
European fission source
One of the most interesting scientific mega-tools under construction today is the European Spallation Source (ESS), which is currently being built in the Swedish city of Lund. This accelerating neutron source is one of the “magnificent four” of new facilities related to neutron physics: MBIR , JHR reactors and IFMIF / EVEDA and ESS accelerator reactors .

One of the architectural concepts of the ESS lab building
But unlike the three previous ones that study the properties of matter in high-power neutron flux as applied to nuclear and thermonuclear technologies, ESS aims to use neutrons for a subtle study of the properties of matter. Cold and ultracold neutrons are a fantastically powerful probing tool - devoid of charge, they easily penetrate the sample, and sophisticated methods for detecting and processing information allow us to study many static and dynamic phenomena at the atomic level.
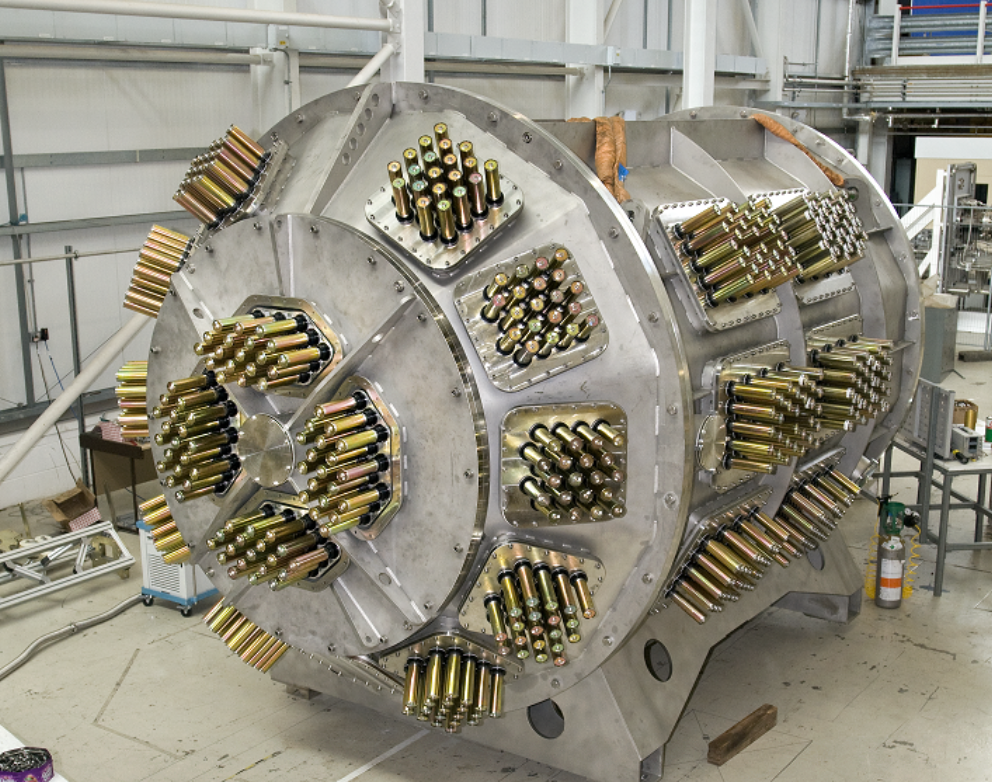
Neutron diffractometer of a similar ISIS accelerator source. Yellow tubes are neutron detectors that measure neutron fluxes.
Today we will talk mainly about the engineering side of ESS and the applications of this latest acquisition of neutron physics.
First of all, back to the title “European splitting (salting) source”. Physicists call salting a process when an energetic particle “cleaves” a handful of protons and neutrons from a target atom. The result of such cleaving or splitting is a powerful neutron flux (protons are inhibited in the target material). This method allows you to get neutron brightness ten times higher than in the most powerful reactors, as well as some chips like profiling the power of a stream in time and its discontinuity, which sometimes physicists need.

Different applications of neutrons as probing radiation.
What can such a source give? The fact is that neutrons are a unique probe for studying matter on a nanoscale. These are not charged particles, which means they easily penetrate into any material - be it a light polymer or heavy steel. However, neutrons are scattered due to interaction with the nucleus of atoms and magnetic moments, which means that the observation of diffraction patterns allows us to understand the internal structure of complex crystals, polymers and other regular structures. Neutron spectrometry allows you to track dynamic processes - for example, many biomolecular processes, the reaction of materials to mechanical load on the scale of atomic ensembles, electrochemical processes in, say, lithium-ion batteries, and even such unexpected things as thermochemical processes in the welding process. In addition, the neutron has a magnetic moment and, according to the polarization of neutrons, one can observe many magneto-quantum effects - for example, the transition to the superconducting state, spin phenomena, quantum liquids, etc. Today, neutron physics is routinely used in applied research in molecular biology, the creation of new materials, the improvement of the properties of batteries and storage systems, semiconductor technology and the development of new superconductors. In this case, mainly thermal, cold, and ultracold neutrons are used. improving the properties of batteries and storage systems, semiconductor technology and the development of new superconductors. In this case, mainly thermal, cold, and ultracold neutrons are used. improving the properties of batteries and storage systems, semiconductor technology and the development of new superconductors. In this case, mainly thermal, cold, and ultracold neutrons are used.

An example of a neutron study of the distribution of elements in a working lithium-ion battery.
Finally, the “neutron activation analysis”, a non-destructive method that makes it possible to finely determine the composition of the sample and the spatial distribution of impurities in it due to the activation (conversion into a radioactive isotope upon absorption of a neutron) of atoms and subsequent gamma-ray spectroscopic investigation of their decay, is slightly distant from the above.
The need for a new high-tech neutron source in Europe is explained by both the obsolescence of the reactors built in the 60s and 70s, and their purely physical aging due to their failure.

Different sources of neutrons: green radioisotope, blue reactor and red accelerator.
Now let's look at the engineering essence of ESS: the
scientific complex is divided into 3 parts - a laboratory with 22 positions for scientific instruments with extracted neutron beams, the Monolith target complex, where neutrons are generated, cooled and distributed, and the Linak accelerator accelerating protons to the target where they “chip” neutrons.

In the middle of the ESS complex rendering, a 600-meter linear accelerator stretched, resting on the right against the target-laboratory buildings
The ESS linear accelerator operates in a pulsed mode, accelerating the clot of protons 14 times per second. The proton energy at the output is 2 GeV, and the accelerator power at the moment of passage of the bunch can reach 125 megawatts (on average, it turns out only 5 megawatts of energy in the beam and 19 consumed by the accelerator itself). The currently established scheme for constructing such facilities implies separation into an ion source, a preparatory “warm” part, and a superconducting main accelerator. The superconducting part is needed to create a greater intensity of the accelerating field - because as the acceleration accelerates, protons pass through the installation faster and less time for energy transfer to them. The ESS accelerator is a 602-meter-long vacuum tube on which accelerating, focusing and controlling elements are worn.

Construction site ESS. In the middle of the frame you can see the long tunnel where the accelerator and the building of microwave energy sources and the accelerator
cryocomplex will be located. The next focusing structure of The Medium Energy Beam Transport (MEBT) 3.9 meters long matches the accelerator section using the classical technology of The Drift Tube Linac drift tubes ( DTL). Here, the proton energy increases from 3.6 to 90 MeV, and the length of this part is 32 meters. Acceleration also occurs by an electromagnetic field.

A characteristic view of a linear accelerator with drift tubes.
Actually, all modern “large” accelerators use the same method of accelerating charged particles with a phased electromagnetic field, which is excited by powerful radio tubes - most often klystrons. However, there are a lot of structures that transmit the energy of the field of directly accelerated matter, and the main thing is not to get confused in them.

For example, such two-spoke resonators will be used in the first stage of the superconducting part of the ESS accelerator.
After gaining sufficient energy in the first part of the accelerator, protons pass into a system of superconducting niobium resonators divided into several groups with increasing frequency. Superconductivity allows you to achieve the highest electromagnetic field strengths with small losses. It is in superconducting modules that the main acceleration occurs - from 90 to 2000 MeV. Resonators are cavities of complex shape of several types, in which an electromagnetic field of very high tension (up to 40 megavolt / meter) is concentrated, maintaining the accelerating potential at the level of 15-20 MeV / meter of the accelerator.

A vacuum cryostat in which similar resonators and auxiliary instruments for measuring the quality of a proton beam are located
Two-band and elliptical resonators are connected to groups of pulsed klystrons with a peak power of about 140 megawatts and the whole system is tuned with nanosecond accuracy to create an accurate distribution of electromagnetic fields along the linac. After the accelerator passes, each proton bunch has an energy close to 2000 MeV, a duration of about 1 millisecond, a pulse current of 62 mA and a repetition rate of 14 hertz (i.e., protons are 1 ms out of every 71.4).

The general layout of the ESS elements and their cost.
This bunch at a speed close to the speed of light crashes into a special target - a 4-ton tungsten wheel with a diameter of 2.5 meters, consisting of 36 lobes and rotating at such a speed that every next proton momentum falls into the next lobule (i.e. about 1 revolution in 2.5 seconds).

Target Wheel ESS. By the way, much more often the target of such a source is in the form of a reservoir with liquid metal.
Protons split tungsten nuclei giving rise to a powerful wave of flying debris, protons, neutrons, muons, etc. etc. Charged particles are decelerated in the wheel itself (the thermal load on the target is almost 5 megawatts, therefore it has a complex cooling system with gaseous helium) and the surrounding steel-concrete bioprotection weighing 6,000 tons, called a “monolith”. Only neutrons passing almost freely through matter are captured by a reflective-retarding system located above and below the wheel.

The reflective-retarding system can be removed from the point where the beam arrives at the target to replace the tungsten wheel (once every 5 years, as the material degrades).
This is the key system of the complex - it is it that works with the neutron “flashlight”, which all ESS instruments look at. In a specially designed cavity of the beryllium reflector there are cavities of a water pre-moderator and the main moderator with liquid hydrogen at a temperature of 20 K. The moderator

located in the drums above and under the wheel. Blue is water, blue is liquid hydrogen. The cross in the center is the source of neutrons.
“Hot” neutrons initially give their momentum to the hydrogen nuclei (in water or hydrogen molecules) cooling to temperatures of several tens of Kelvin. The lower the speed, the longer the wavelength and the greater the uncertainty of position. The neutrons are cooled so that their wavelength increases and becomes comparable with the distance between the atoms in the sample and the diffraction pattern becomes distinguishable. And even after cooling with liquid hydrogen for most neutrons, the wavelength is too short, so you have to select only that small fraction that relates to the “tail” of the Maxwell distribution with ultra-low energies (thanks antihydrogen for clarification). Actually at such temperatures, the wavelength and resolution of the neutron image is tenths of a nanometer, i.e. in fact, in the limit are single atoms.
Four tanks with a hydrogen moderator are formed by 4 neutron sources, which are output through sets of neutron guides (which are steel pipes with a multilayer inner coating of a special shape, operating in fact by mirror optics).

Neutron output lines from a reflective-decelerating system.
From the focal points of the moderator, 42 neutron guides fan out, 22 of which will be used in the first phase of the laboratory, and another 20 are left for further upgrades. Neutron guides are up to 160 meters long and pass through many devices: shutters that provide the necessary “shutter speed”, rotating choppers, cut the profile and provide a stroboscopic mode for dynamic measurements, and monochromators that overlap the neutron guide in series so that only neutrons reach the sample and device one speed (= energy, i.e. providing the release of a certain spectrum of neutrons).
A good idea of the operation of a typical station with neutron optics and a detector that immediately determines the spatial, amplitude, and spectral distribution of neutrons interacting with the sample giveshere is this interactive picture .

Together. At the top left is a “monolith” with proton input (left) and neutron exit (right), below a neutron guide with optics and bioprotection.

And this is what neutron guides look like.
Currently, 16 instruments are selected for 22 positions, which are grouped by research methods. I think it will be more interesting to look specifically at the research methods.

ESS neutron complex in buildings (the second half of the building is not shown).
In general, work at such facilities reduces to the interaction of the prepared neutron flux with the sample and to the study of the spatial, amplitude, and spectral distribution of neutrons interacting with the sample. The simplest option is a neutron camera, similar to an x-ray machine in principle. In the ESS lab, this tool is ODIN. It uses the principle of a pinhole camera, is armed with monochromators and polarizers to obtain images in a neutron beam of different energy and polarization and has an extremely high spatial resolution (units of microns per pixel). The main focus of the device is biology, distribution and transport of hydrogen in samples, however, in engineering, the device is also able to be beneficial - for example, using it, material science can be observed during the welding of steel.

ODIN in the protective hopper ...

And the tool itself is blocky. On the left is a pinhole collimator, on the right is a camera.
The second important methodological approach is the study of neutron diffraction on a regular crystal structure, as well as small-angle scattering on films - both of these methods can restore the spatial arrangement of atoms in the molecules of the studied crystal or film from the resulting brightness peaks. For these tasks, ESS will immediately use a mass of SKADI and LoKI tools for studying films and surfaces by small angle scattering, MAGiC for studying neutron diffraction on single crystals, HEIMDAL and DREAM for powder diffraction, NMX for studying diffraction on crystals of large biological molecules (for example, restoration of the structure of protein molecules, as is done by x-ray analysis).

NMX will use robotic arms to move the detectors.
BEER tool for studying at the same time the microcrystalline structure of materials and their behavior during work with the analysis of characteristic interaction energies. This is useful for obtaining new fiber foundations of composite materials, new metal alloys, as well as research in the field of battery materials.



Often neutron instruments are equipped with cryo-vacuum sample vessels. They use high-tech sample holders in their own way.
The next group of devices are reflectometers that study the reflection of neutrons from the surfaces of samples. For ESS, these are FREIA and ESTIA. This technique is used to obtain information on the density, thickness, roughness and magnetic properties of thin coatings and films, up to atomic thickness. In addition to obvious applications in engineering, such tools are used in the study of biological membranes, for example, FREIA will work with films on the surface of a liquid.

An example of a small-angle reflectometer device working with films on liquid. The neutron input and optics are on the right, the sample positioner is to the left of the center, and the detector unit is on the left.
Finally, the final review technique - spectrometric studies is represented by the largest number of instruments. Spectroscopy allows you to study the dynamics and kinetics of atoms in a sample, due to the fact that incident neutrons have approximately the same energy as the energy of bonds between atoms in crystals and biological molecules. Spectroscopy in neutron technology is direct when the sample is transmitted through neutrons with a gradually changing wavelength and back when a “white” neutron source is used and the spectral response is calculated from the time of neutron movement from the sample to the detectors. Direct spectrometers at ESS are VOR, C-SPEC (with cold neutron optics), T-REX, and MIRACLES instruments.
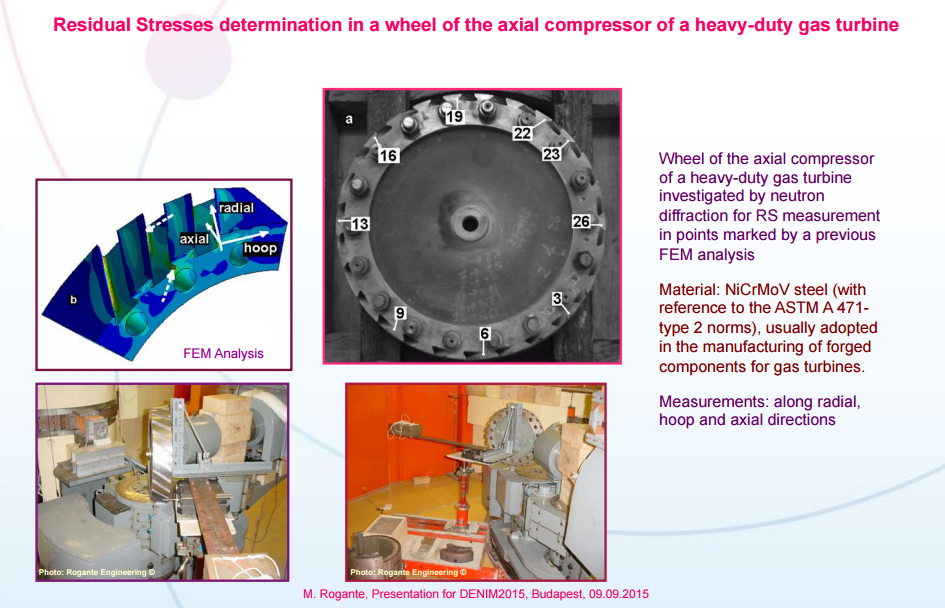
And here is an example of using neutron diffractometers to study the residual tension in a gas turbine disk.
More complex spectroscopes of inverse geometry will be presented by BIFROST and VESPA instruments. The latter specializes in the study of vibrational modes of molecules, which is very interesting for the search for new high-energy substances - fuels, explosives, battery chemistry.
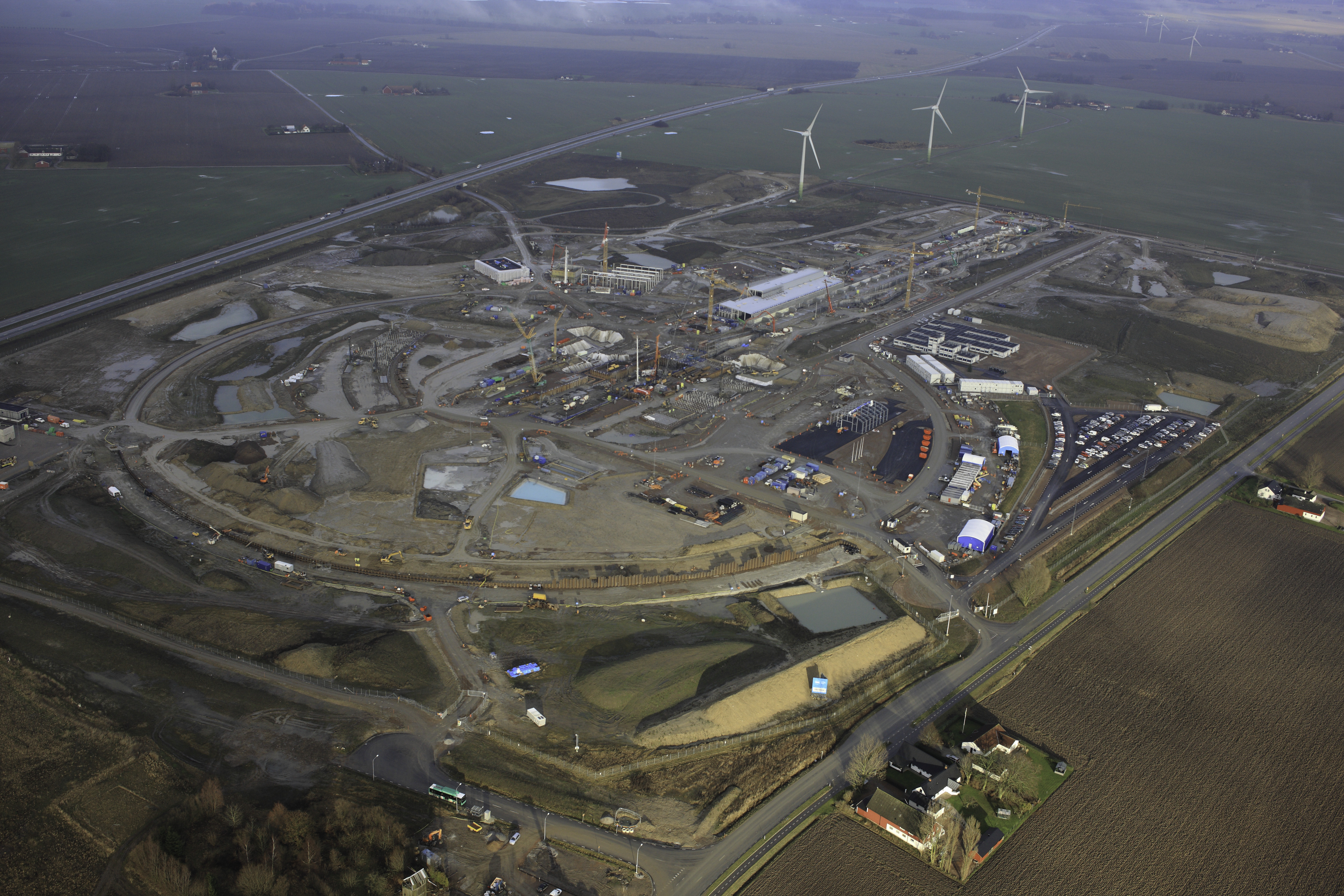
Construction of a European spa source at the end of 2015
The magnificent complex of neutron physics ESS is now at the stage of building construction, and construction will last at least another two years. Only at the beginning of 2018 will the installation of the main equipment begin, and from 2019 it is planned to phase in the first accelerator, then the target and, starting in 2020, scientific instruments. By 2023, the complex should start operating normally, providing hundreds of experiments every year.

One of the architectural concepts of the ESS lab building
But unlike the three previous ones that study the properties of matter in high-power neutron flux as applied to nuclear and thermonuclear technologies, ESS aims to use neutrons for a subtle study of the properties of matter. Cold and ultracold neutrons are a fantastically powerful probing tool - devoid of charge, they easily penetrate the sample, and sophisticated methods for detecting and processing information allow us to study many static and dynamic phenomena at the atomic level.
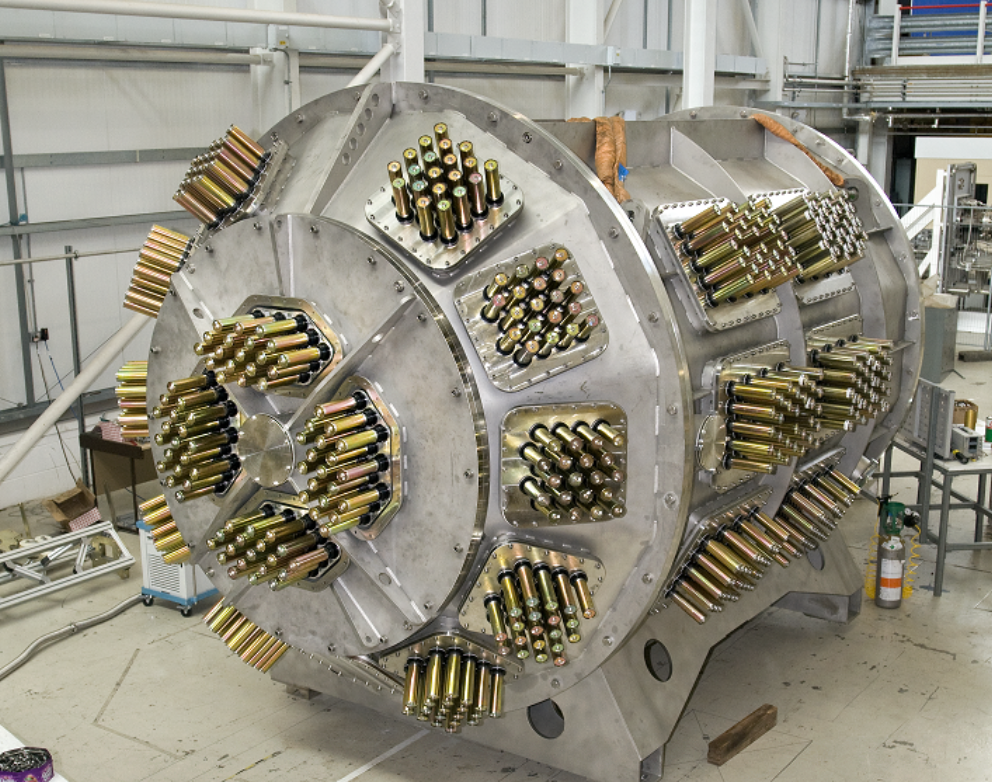
Neutron diffractometer of a similar ISIS accelerator source. Yellow tubes are neutron detectors that measure neutron fluxes.
Today we will talk mainly about the engineering side of ESS and the applications of this latest acquisition of neutron physics.
First of all, back to the title “European splitting (salting) source”. Physicists call salting a process when an energetic particle “cleaves” a handful of protons and neutrons from a target atom. The result of such cleaving or splitting is a powerful neutron flux (protons are inhibited in the target material). This method allows you to get neutron brightness ten times higher than in the most powerful reactors, as well as some chips like profiling the power of a stream in time and its discontinuity, which sometimes physicists need.

Different applications of neutrons as probing radiation.
What can such a source give? The fact is that neutrons are a unique probe for studying matter on a nanoscale. These are not charged particles, which means they easily penetrate into any material - be it a light polymer or heavy steel. However, neutrons are scattered due to interaction with the nucleus of atoms and magnetic moments, which means that the observation of diffraction patterns allows us to understand the internal structure of complex crystals, polymers and other regular structures. Neutron spectrometry allows you to track dynamic processes - for example, many biomolecular processes, the reaction of materials to mechanical load on the scale of atomic ensembles, electrochemical processes in, say, lithium-ion batteries, and even such unexpected things as thermochemical processes in the welding process. In addition, the neutron has a magnetic moment and, according to the polarization of neutrons, one can observe many magneto-quantum effects - for example, the transition to the superconducting state, spin phenomena, quantum liquids, etc. Today, neutron physics is routinely used in applied research in molecular biology, the creation of new materials, the improvement of the properties of batteries and storage systems, semiconductor technology and the development of new superconductors. In this case, mainly thermal, cold, and ultracold neutrons are used. improving the properties of batteries and storage systems, semiconductor technology and the development of new superconductors. In this case, mainly thermal, cold, and ultracold neutrons are used. improving the properties of batteries and storage systems, semiconductor technology and the development of new superconductors. In this case, mainly thermal, cold, and ultracold neutrons are used.

An example of a neutron study of the distribution of elements in a working lithium-ion battery.
Finally, the “neutron activation analysis”, a non-destructive method that makes it possible to finely determine the composition of the sample and the spatial distribution of impurities in it due to the activation (conversion into a radioactive isotope upon absorption of a neutron) of atoms and subsequent gamma-ray spectroscopic investigation of their decay, is slightly distant from the above.
The need for a new high-tech neutron source in Europe is explained by both the obsolescence of the reactors built in the 60s and 70s, and their purely physical aging due to their failure.

Different sources of neutrons: green radioisotope, blue reactor and red accelerator.
Now let's look at the engineering essence of ESS: the
scientific complex is divided into 3 parts - a laboratory with 22 positions for scientific instruments with extracted neutron beams, the Monolith target complex, where neutrons are generated, cooled and distributed, and the Linak accelerator accelerating protons to the target where they “chip” neutrons.

In the middle of the ESS complex rendering, a 600-meter linear accelerator stretched, resting on the right against the target-laboratory buildings
The ESS linear accelerator operates in a pulsed mode, accelerating the clot of protons 14 times per second. The proton energy at the output is 2 GeV, and the accelerator power at the moment of passage of the bunch can reach 125 megawatts (on average, it turns out only 5 megawatts of energy in the beam and 19 consumed by the accelerator itself). The currently established scheme for constructing such facilities implies separation into an ion source, a preparatory “warm” part, and a superconducting main accelerator. The superconducting part is needed to create a greater intensity of the accelerating field - because as the acceleration accelerates, protons pass through the installation faster and less time for energy transfer to them. The ESS accelerator is a 602-meter-long vacuum tube on which accelerating, focusing and controlling elements are worn.

Construction site ESS. In the middle of the frame you can see the long tunnel where the accelerator and the building of microwave energy sources and the accelerator
cryocomplex will be located. The next focusing structure of The Medium Energy Beam Transport (MEBT) 3.9 meters long matches the accelerator section using the classical technology of The Drift Tube Linac drift tubes ( DTL). Here, the proton energy increases from 3.6 to 90 MeV, and the length of this part is 32 meters. Acceleration also occurs by an electromagnetic field.

A characteristic view of a linear accelerator with drift tubes.
Actually, all modern “large” accelerators use the same method of accelerating charged particles with a phased electromagnetic field, which is excited by powerful radio tubes - most often klystrons. However, there are a lot of structures that transmit the energy of the field of directly accelerated matter, and the main thing is not to get confused in them.

For example, such two-spoke resonators will be used in the first stage of the superconducting part of the ESS accelerator.
After gaining sufficient energy in the first part of the accelerator, protons pass into a system of superconducting niobium resonators divided into several groups with increasing frequency. Superconductivity allows you to achieve the highest electromagnetic field strengths with small losses. It is in superconducting modules that the main acceleration occurs - from 90 to 2000 MeV. Resonators are cavities of complex shape of several types, in which an electromagnetic field of very high tension (up to 40 megavolt / meter) is concentrated, maintaining the accelerating potential at the level of 15-20 MeV / meter of the accelerator.

A vacuum cryostat in which similar resonators and auxiliary instruments for measuring the quality of a proton beam are located
Two-band and elliptical resonators are connected to groups of pulsed klystrons with a peak power of about 140 megawatts and the whole system is tuned with nanosecond accuracy to create an accurate distribution of electromagnetic fields along the linac. After the accelerator passes, each proton bunch has an energy close to 2000 MeV, a duration of about 1 millisecond, a pulse current of 62 mA and a repetition rate of 14 hertz (i.e., protons are 1 ms out of every 71.4).

The general layout of the ESS elements and their cost.
This bunch at a speed close to the speed of light crashes into a special target - a 4-ton tungsten wheel with a diameter of 2.5 meters, consisting of 36 lobes and rotating at such a speed that every next proton momentum falls into the next lobule (i.e. about 1 revolution in 2.5 seconds).

Target Wheel ESS. By the way, much more often the target of such a source is in the form of a reservoir with liquid metal.
Protons split tungsten nuclei giving rise to a powerful wave of flying debris, protons, neutrons, muons, etc. etc. Charged particles are decelerated in the wheel itself (the thermal load on the target is almost 5 megawatts, therefore it has a complex cooling system with gaseous helium) and the surrounding steel-concrete bioprotection weighing 6,000 tons, called a “monolith”. Only neutrons passing almost freely through matter are captured by a reflective-retarding system located above and below the wheel.

The reflective-retarding system can be removed from the point where the beam arrives at the target to replace the tungsten wheel (once every 5 years, as the material degrades).
This is the key system of the complex - it is it that works with the neutron “flashlight”, which all ESS instruments look at. In a specially designed cavity of the beryllium reflector there are cavities of a water pre-moderator and the main moderator with liquid hydrogen at a temperature of 20 K. The moderator

located in the drums above and under the wheel. Blue is water, blue is liquid hydrogen. The cross in the center is the source of neutrons.
“Hot” neutrons initially give their momentum to the hydrogen nuclei (in water or hydrogen molecules) cooling to temperatures of several tens of Kelvin. The lower the speed, the longer the wavelength and the greater the uncertainty of position. The neutrons are cooled so that their wavelength increases and becomes comparable with the distance between the atoms in the sample and the diffraction pattern becomes distinguishable. And even after cooling with liquid hydrogen for most neutrons, the wavelength is too short, so you have to select only that small fraction that relates to the “tail” of the Maxwell distribution with ultra-low energies (thanks antihydrogen for clarification). Actually at such temperatures, the wavelength and resolution of the neutron image is tenths of a nanometer, i.e. in fact, in the limit are single atoms.
Four tanks with a hydrogen moderator are formed by 4 neutron sources, which are output through sets of neutron guides (which are steel pipes with a multilayer inner coating of a special shape, operating in fact by mirror optics).

Neutron output lines from a reflective-decelerating system.
From the focal points of the moderator, 42 neutron guides fan out, 22 of which will be used in the first phase of the laboratory, and another 20 are left for further upgrades. Neutron guides are up to 160 meters long and pass through many devices: shutters that provide the necessary “shutter speed”, rotating choppers, cut the profile and provide a stroboscopic mode for dynamic measurements, and monochromators that overlap the neutron guide in series so that only neutrons reach the sample and device one speed (= energy, i.e. providing the release of a certain spectrum of neutrons).
A good idea of the operation of a typical station with neutron optics and a detector that immediately determines the spatial, amplitude, and spectral distribution of neutrons interacting with the sample giveshere is this interactive picture .

Together. At the top left is a “monolith” with proton input (left) and neutron exit (right), below a neutron guide with optics and bioprotection.

And this is what neutron guides look like.
Currently, 16 instruments are selected for 22 positions, which are grouped by research methods. I think it will be more interesting to look specifically at the research methods.

ESS neutron complex in buildings (the second half of the building is not shown).
In general, work at such facilities reduces to the interaction of the prepared neutron flux with the sample and to the study of the spatial, amplitude, and spectral distribution of neutrons interacting with the sample. The simplest option is a neutron camera, similar to an x-ray machine in principle. In the ESS lab, this tool is ODIN. It uses the principle of a pinhole camera, is armed with monochromators and polarizers to obtain images in a neutron beam of different energy and polarization and has an extremely high spatial resolution (units of microns per pixel). The main focus of the device is biology, distribution and transport of hydrogen in samples, however, in engineering, the device is also able to be beneficial - for example, using it, material science can be observed during the welding of steel.

ODIN in the protective hopper ...

And the tool itself is blocky. On the left is a pinhole collimator, on the right is a camera.
The second important methodological approach is the study of neutron diffraction on a regular crystal structure, as well as small-angle scattering on films - both of these methods can restore the spatial arrangement of atoms in the molecules of the studied crystal or film from the resulting brightness peaks. For these tasks, ESS will immediately use a mass of SKADI and LoKI tools for studying films and surfaces by small angle scattering, MAGiC for studying neutron diffraction on single crystals, HEIMDAL and DREAM for powder diffraction, NMX for studying diffraction on crystals of large biological molecules (for example, restoration of the structure of protein molecules, as is done by x-ray analysis).

NMX will use robotic arms to move the detectors.
BEER tool for studying at the same time the microcrystalline structure of materials and their behavior during work with the analysis of characteristic interaction energies. This is useful for obtaining new fiber foundations of composite materials, new metal alloys, as well as research in the field of battery materials.



Often neutron instruments are equipped with cryo-vacuum sample vessels. They use high-tech sample holders in their own way.
The next group of devices are reflectometers that study the reflection of neutrons from the surfaces of samples. For ESS, these are FREIA and ESTIA. This technique is used to obtain information on the density, thickness, roughness and magnetic properties of thin coatings and films, up to atomic thickness. In addition to obvious applications in engineering, such tools are used in the study of biological membranes, for example, FREIA will work with films on the surface of a liquid.

An example of a small-angle reflectometer device working with films on liquid. The neutron input and optics are on the right, the sample positioner is to the left of the center, and the detector unit is on the left.
Finally, the final review technique - spectrometric studies is represented by the largest number of instruments. Spectroscopy allows you to study the dynamics and kinetics of atoms in a sample, due to the fact that incident neutrons have approximately the same energy as the energy of bonds between atoms in crystals and biological molecules. Spectroscopy in neutron technology is direct when the sample is transmitted through neutrons with a gradually changing wavelength and back when a “white” neutron source is used and the spectral response is calculated from the time of neutron movement from the sample to the detectors. Direct spectrometers at ESS are VOR, C-SPEC (with cold neutron optics), T-REX, and MIRACLES instruments.
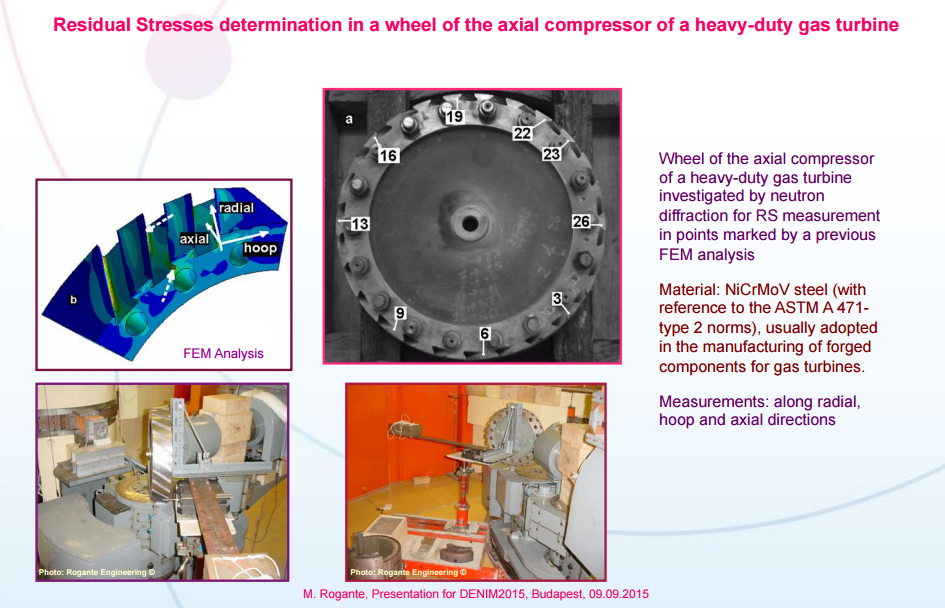
And here is an example of using neutron diffractometers to study the residual tension in a gas turbine disk.
More complex spectroscopes of inverse geometry will be presented by BIFROST and VESPA instruments. The latter specializes in the study of vibrational modes of molecules, which is very interesting for the search for new high-energy substances - fuels, explosives, battery chemistry.
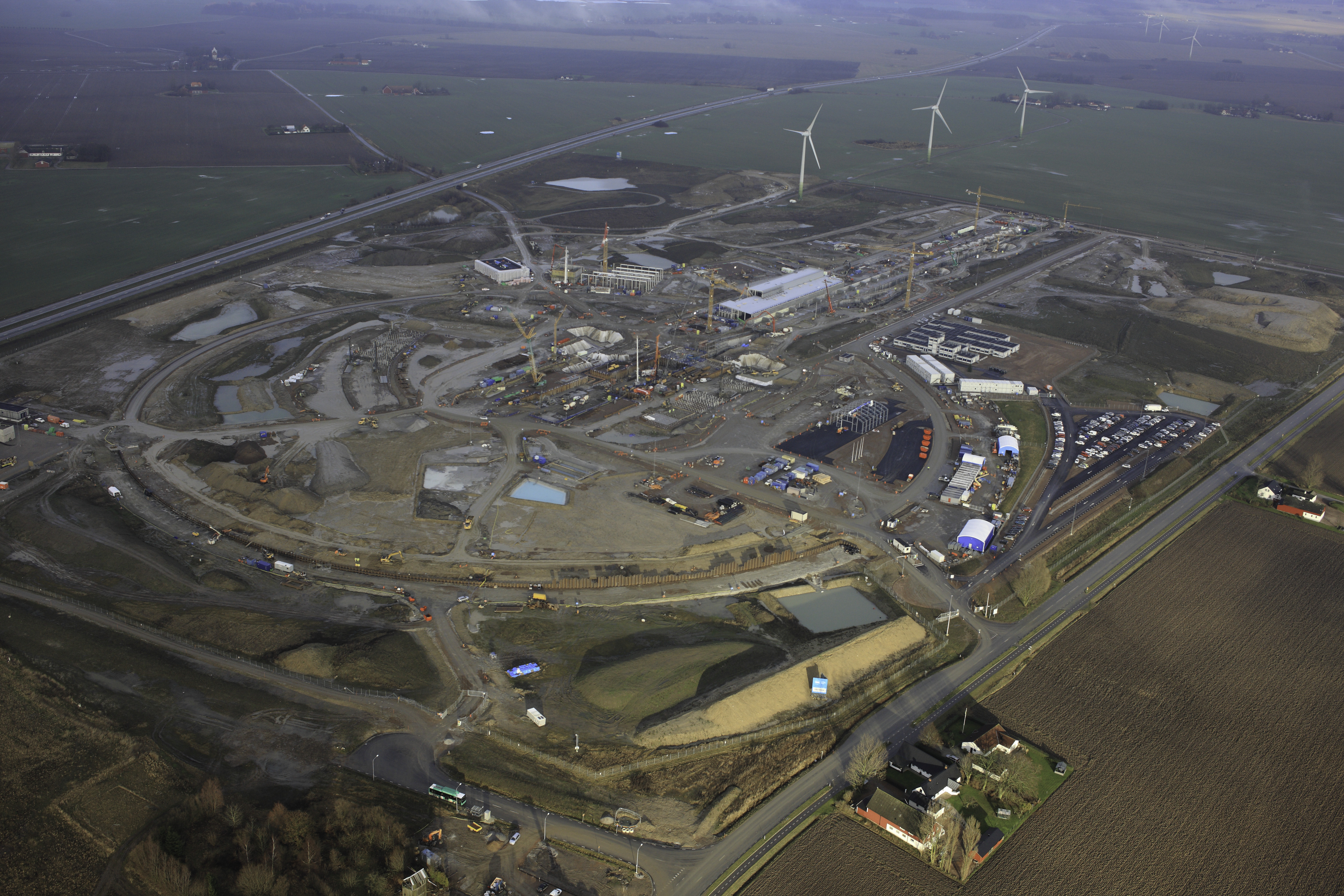
Construction of a European spa source at the end of 2015
The magnificent complex of neutron physics ESS is now at the stage of building construction, and construction will last at least another two years. Only at the beginning of 2018 will the installation of the main equipment begin, and from 2019 it is planned to phase in the first accelerator, then the target and, starting in 2020, scientific instruments. By 2023, the complex should start operating normally, providing hundreds of experiments every year.