A brief history of the physical theory of the great unification
- Transfer
Lawrence Kraus is a theoretical physicist, cosmologist, director of the Origins project, and founder of the Earth and Space Exploration School at Arizona State University. He is the author of such best-selling books as A Universe from Nothing [A Universe from Nothing] and Star Trek Physics [The Physics of Star Trek]. Translation of an excerpt from his future book, The Greatest Story Told So far: Why Are We Here? [The Greatest Story Ever Told — So Far: Why Are We Here?].
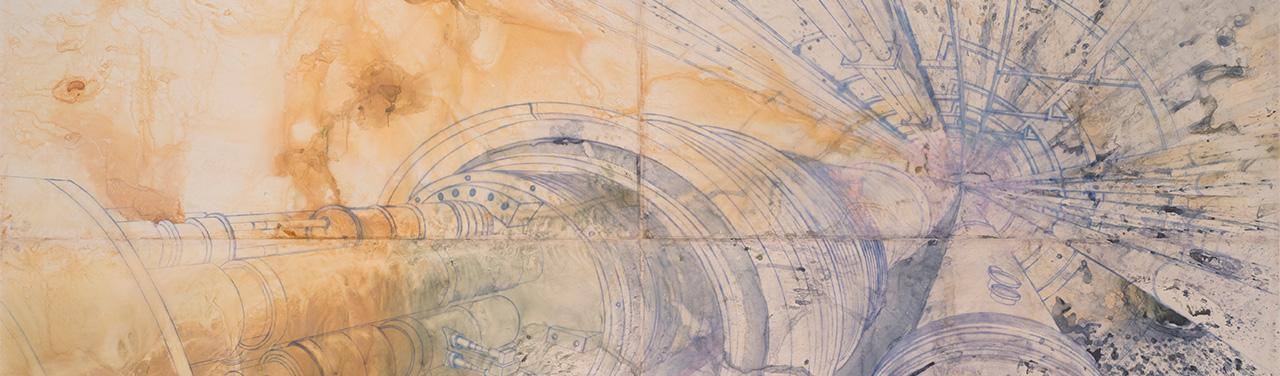
Specialists in particle physics before the discovery of the Higgs particle in 2012 dreamed of two types of nightmares. The first is that the Large Hadron Collider (LHC) will not find anything. In this case, it would be the last major accelerator built to probe the fundamental structure of the universe. The second is that the Higgs particle predicted by theoretical physicist Peter Higgs in 1964 will be found at the LHC ... and nothing more.
Each discovery of one level of reality shows us the following levels. Therefore, each important discovery in science usually leaves us with more questions than answers. But on the other hand, it usually gives us at least an outline of a further path, helping us to find answers to new questions. The successful discovery of the Higgs particle and the confirmation of the existence in space of an invisible background of the Higgs field (in the quantum world, each particle, such as the Higgs particle, is associated with the field), was a powerful confirmation of the bold scientific discoveries of the 20th century.

But the words of Sheldon Lee Glashow have not lost their relevance: the Higgs particle is similar to the sewer. She hides all the untidy details that we don’t want to talk about. The Higgs field interacts with most elementary particles when they move in space, and creates a resistance force that slows their movement and gives them the appearance of mass. Therefore, the masses of elementary particles, measured by us, and making possible our familiar world, are something like an illusion, an accident of our perception.
This idea may look elegant, but in reality it represents a special addition to the Standard Model of Physics - explaining three of the four known forces, and how they interact with matter. It was added to the theory in order to satisfy the requirements for an accurate description of our world. But the theory itself does not require it. The universe could easily exist with massless particles and long-range weak interactions (one of the four interactions — the rest will be strong, electromagnetic, and gravitational force). Just there would not be us and our questions. Moreover, the exact physics of the Higgs model is not defined within the Standard Model alone. A particle could be 20 times heavier or 100 times lighter.
So why does it even exist? And why is she so heavy? (Considering that when a scientist asks the question “Why?”, In fact he means “How?”) If there were no Higgs particles, there would not be such a world as we observe - but this is clearly not possible an explanation. Or can it? To understand the basis of Higgs physics is to understand how we appeared. When we ask: “Why are we here?”, In essence, we ask: “Why is the Higgs here?” And the Standard Model cannot answer this question.
There are some hints derived from a combination of theory and experiment. Shortly after establishing the clear structure of the Standard Model, in 1974, and long before experimentally confirming its details in the next decade, two different groups of Harvard physicists, in which Sheldon Lee Glashow worked, andSteven Weinberg , noticed something interesting. Glashow, together with Howard Georgie , did what he could best: he looked for patterns in existing particles and interactions, and new possibilities with the help of the mathematical theory of groups.
In the Standard Model, weak and electromagnetic interactions are combined at high energies into a single force, which physicists call "electro-weak." This means that the same mathematics controls the weak and electromagnetic interaction, they both obey the same symmetries, and these two forces are different reflections of the same unified theory. But the symmetry is “spontaneously broken” by the Higgs field interacting with particles that undergo weak interaction, but not with particles that transfer electromagnetic. This property of nature leads to the fact that these two interactions look separate and differ on the scales available to our measurements - while the weak interaction works at short distances, and the electromagnetic interaction - at long distances.
Georgie and Glashow attempted to expand this idea and connect strong interactions to them, and found that all known particles and three non-gravitational interactions fit naturally in one fundamentally symmetric structure. They reasoned that this symmetry can spontaneously break down on some ultra-high energy scale (and at a short distance), beyond the limits of the possibilities of modern experiments, and generate two separate symmetries — strong and electro-weak interaction. As a result, at lower energies and at large distances electroweak symmetry is destroyed, dividing the electroweak interaction into weak, acting at short distances, and electromagnetic, acting at long distances.
Such a theory they modestly called the theory of the great unification (TVO).
Around the same time, Weinberg and Georgie, along with Helen Quinn, noticed something interesting in developing the work of Frank Wilcheck, David Gross and David Politzer. If at small distances the strong interaction becomes weaker, then the electromagnetic and weak ones become stronger.
It was not necessary to be a rocket scientist in order to be interested in whether the strength of three different interactions on some small scale does not coincide. After calculating, they found (with the accuracy with which interactions were measured) that such a combination is possible, but only at distances of 15 orders of magnitude smaller than the size of the proton.
If TVO was the one proposed by Howard Georgie and Glashow - then it was good news, because if all the particles we observe in nature combine in this way, then new particles ( gauge bosons ) must exist that provide the connection between the quarks (of which the protons and neutrons) and electrons with neutrinos. This would mean that protons can decay into lighter particles, which we can observe in principle. As Glashow wrote, "diamonds are not forever."
And even then it was known that the proton lifetime was extremely long. Not only because we still exist 14 billion years after the Big Bang, but also because we don’t die of cancer in childhood. If the average lifetime of a proton would be less than a billion billion years, then in childhood enough protons would decay in our body so that their radiation would kill us. In quantum mechanics, all processes are probabilistic. If the average proton lives a billion billion years, and if you have a billion billion protons, then one of them will decay on average every year. And in our body is much more than a billion billion protons.
However, with such an incredibly small scale of distances, and, therefore, with such a huge mass scale associated with spontaneous symmetry breaking in TVO, the new gauge bosons receive huge masses. And this would lead to the fact that the interactions controlled by them would occur at such small distances that they would be incredibly weak in terms of protons and neutrons. As a result, although the protons may decay, in our case, before that, they can live, perhaps a million billion billion billion years.
Thanks to the results obtained by Glashow with Georgie, as well as Georgie with Quinn and Weinberg, the expectations of the great synthesis were in the air. After the success of the electroweak theory of physics, studying particles, were ambitious and believed in the subsequent unification.
How do you know if these ideas are true? It was impossible to build an accelerator capable of working on energies a million billion times more than the rest mass of protons. The circumference of such a machine would have to be compared with the orbit of the moon. And even if it were possible, as a result of the fiasco of the Superconducting supercollider, no government would approve such an estimate. [This collider, also called the Desertron, should have been built in Texas in the 1990s, but due to problems with the budget was cancelled. It was planned that the length of its circumference will be 87.1 km. $ 2 billion was spent on construction, and the final cost was estimated at $ 12 billion - approx. trans.].
Fortunately, there was another way - to use the probability described by me, which limits the proton lifetime. If TVO predicts a proton lifetime of one thousand billion billion billion years, then you need to push a thousand billion billion billion protons into one detector, and then on average one of them will decay every year.
And where do you get so many protons? It's simple: in 3000 tons of water.
All that was required for that was to place a tank of water in the dark, make sure that there was no radioactive background in this place, surround it with sensitive photocells able to detect flashes of light in the detector, and then wait a year waiting for a flash of light when a proton decays. It sounds scary, but at least two large experimental facilities were paid for and built according to this scheme - one deep underground in the salt mine near Lake Erie (IMB), the other in the Kamioka zinc mine in Japan (Kamiokanda). The mines were used to cut off cosmic rays, against which it would have been impossible to notice the proton decay.

The Large Hadron Collider
Both experiments began to work in 1982-1983. Scientists were so enthusiastic about TVO that they were confidently waiting for the signal to appear soon. In this case, a TSS would be the culmination of a decade of tremendous development and discoveries in particle physics - not to mention the next Nobel Prize for Glashow , and possibly some more.
Unfortunately, in this case, nature was not so good. Not a single signal appeared either in the first year, or in the second, or in the third. The simplest and elegant model of Glashow and Georgie soon had to be rejected. But the fever TVO has already captured scientists, and it was difficult to get rid of it. Other proposals were made about theories of unification, because of which the decay of protons would go beyond the framework of current experiments.
On February 23, 1987, another event happened that again demonstrated an almost universal aphorism: every new window into the Universe takes us by surprise. On that day, a group of astronomers on photographic plates accumulated overnight discovered the nearest exploded star (supernova) of all that we have seen in the last 400 years. This star, which was 160,000 light-years away, was in the Large Magellanic Cloud — a small galaxy, a satellite of the Milky Way, which can be seen in the southern hemisphere.
If our theories about exploding stars are correct, most of the energy they emit should take the form of neutrinos, despite the fact that the light of their explosion is the brightest of cosmic fireworks (and they explode about one star in one galaxy in 100 years). Rough calculations showed that the IMB and Kamiokande water detectors were to detect about 20 collisions with neutrinos. And when the experimenters of these detectors studied the data of that day, 8 candidates were found at IBM on a 10-second interval, and on Kamiokand - 11. For neutrino physics, this was just a sea of data. Neutrino astrophysics has suddenly matured. Probably 1900 scientific works of various physicists (including me) were based on these 19 events. They realized that this event opened an unprecedented window into the nuclei of exploding stars, and into the laboratory not only for astrophysics,
Driven by the idea that large proton decay detectors can simultaneously become astrophysical neutrino detectors, several groups of scientists have begun to build a new generation of such dual-use detectors. The largest was re-built in the Kamioka mine and dubbed Super-Kamiokande - and for good reason. This giant reservoir of water weighing 50,000 tons, surrounded by 11,800 photocells, worked in an operating mine, and the experiment was conducted with laboratory cleanliness. It was necessary, because with such a huge detector it was necessary to take care not only of external cosmic rays, but also of internal radioactive contamination, which would eclipse all useful signals.
Meanwhile, interest in astrophysical neutrinos was also at its peak. The sun emits neutrinos as a result of nuclear reactions in its core, and for 20 years the physicist Ray Davis discovered solar neutrinos, but the events happened three times less often than the best models of the Sun predicted. A new type of solar neutrino detector, called the Sudbury Neutrino Observatory (SNO), was built in the mine town of Sudbury in Canada.
To date, the Super-Kamiokande has almost continuously worked, sometimes undergoing various improvements, for 20 years. No signals from the decay of protons and no new supernovae have been observed since. However, accurate neutrino observations, coupled with additional observations at SNO, unequivocally confirmed the reality of the solar neutrino deficit, discovered by Ray Davis. It was found that the deficit does not exist because of astrophysical phenomena occurring in the Sun, but because of the properties of the neutrino. It became clear that at least one of the three types of neutrinos is not massless. Since the Standard model of the neutrino mass is not included, it was the first confirmed observation that some new physics in nature works outside the Standard Model and the Higgs.
High-energy neutrinos regularly bombard the Earth after protons from high-energy cosmic rays collide with the atmosphere and produce a wide air shower from secondary subatomic particles, where these neutrinos are also encountered. Observations on them showed that the second type of neutrino has a mass. It is slightly larger than the first, but much smaller than the electron mass. For this observation, teams from SNO and Kamiokande received the Nobel Prize in 2015 - a week before I started writing this book. To this day, these seductive hints of new physics have not been explained with the help of our theories.
The lack of proton decay was a disappointment, but not a complete surprise. From the moment when TWR was first proposed, the landscape of physics has changed. More accurate measurements of the values of three non-gravitational interactions, together with more complex calculations of changing their magnitude with distance, showed that if only particles from the Standard Model exist in nature, then the forces of these three interactions will not unite on the same scale. In order for the Great Unification to occur, there will have to be a new physics on an energy scale that surpasses everything we have seen so far. And the presence of new particles would not only change the energy scale for combining the three interactions, but also increase the scale for TVO,

The Large Hadron Collider.
In parallel with these events, theorists actively used new mathematical tools to study a new probable type of symmetry, which they began to call supersymmetry. This fundamental symmetry differs from other previously known ones in that it connects two different types of particles — fermions (particles with a half-integer spin) and bosons (particles with a whole spin). But the point is that if such symmetry is observed in nature, then for every particle known in the Standard Model there must be at least one new particle. For each new boson, a fermion must exist. For each fermion is a boson.
Since we do not observe these particles, this symmetry cannot manifest itself at the level of the universe accessible to us, and, therefore, must be broken - and this means that new particles must have masses large enough for them not to be found in existing accelerators.
What is so attractive about symmetry, which suddenly doubles the number of particles in nature, when there is no evidence of their existence? For the most part, she seduces with Great Unification. Since if TVO exists on the scale of masses by 15-16 orders of magnitude of a large rest mass of a proton, then it is 13 orders of magnitude larger than the scales on which electroweak symmetry is broken. The question is how and why in the fundamental laws of nature there is such a huge scale gap. In particular, if the Higgs is really the last particle of the Standard Model, then the question arises: why is the energy scale of the violation of the Higgs symmetry by 13 orders of magnitude smaller than the scale of violation of the symmetry of some new field that breaks the TVO symmetry into separate interactions?
And the problem is even worse than it seems. If we consider the effects of virtual particles (arising and disappearing so quickly that their existence can only be confirmed indirectly), including particles of arbitrarily large mass (such as the gauge particles of the proposed TVO), it becomes clear that the mass and scale of the Higgs symmetry violation increase so that approaching the huge scale of TVO. From here comes the so-called. the problem of naturalness . Technically speaking, there is an unnatural existence of a huge hierarchy of scales , from those on which the Higgs particle breaks electroweak symmetry, and to those on which the symmetry of TVO is broken by a new scalar field, whatever that may be.
Mathematical physicist Edward Whitten wrote in his landmark work of 1981 that supersymmetry has a special property. It can reduce the effect of virtual particles of arbitrarily large mass and energy on the properties of the world on the scales that are available for our observations. Since virtual fermions and virtual bosons of the same mass lead to identical, not counting the sign, quantum corrections, if each boson corresponds to a fermion of the same mass, then the quantum effects of virtual particles are mutually destroyed. And this means that the effect of virtual particles of arbitrarily large mass and energy on the properties of the world on the scales that are available for our observations completely disappear.
If supersymmetry itself is broken (as it should be, otherwise all supersymmetric partners of ordinary matter would have the same mass as ordinary particles, and we would have discovered them long ago), then quantum corrections are not destroyed. They lead to additions to masses on scales comparable to those on which supersymmetry is broken. If they were comparable to the scale of breaking of electroweak symmetry, this would explain the scale of the Higgs mass.
This also means that we can expect to see a heap of new particles — supersymmetric partners of ordinary matter — on the scales that the LHC is now probing.
This would solve the problem of naturalness, since it would protect the Higgs boson masses from quantum corrections that would inflate them to the energy scale of the TWR. Supersymmetry can allow the existence of a “naturally” large hierarchy of energy and mass, which separates the electro-weak scale and the scale of TVO.
The fact that supersymmetry can in principle solve the problem of hierarchy has increased its appeal to physicists. Theorists began to explore realistic models that included the violation of supersymmetry and other consequences of this idea. After that, the “market value” of supersymmetry broke all records. Since, if we include the possibility of spontaneous supersymmetry breaking in the calculations of the change in three non-gravitational interactions depending on the distance, it turns out that the strength of these three interactions naturally suddenly converges to one scale of small distances. TVO returned to duty!
The models with broken supersymmetry have another attractive feature. Long before the discovery of the upper quark, it was shown that if the upper quark is heavy, then through interaction with other supersymmetric partners it can contribute to quantum corrections to the properties of the Higgs particle, which will lead to a change in the Higgs field. If the Grand Unification happens on a much larger energy scale, then the Higgs field should form a coherent background throughout the space at the energy level at which the current measurements are taken. In short, the energy scale of the electroweak symmetry breaking can naturally occur in a theory in which VO happens on a much larger scale. And when the top quark was discovered and found that it was really heavy,
But all this has a price. For a theory to work, there must be two Higgs bosons, not just one. Moreover, in such an accelerator as the LHC, it would be necessary to find new supersymmetric particles — it can probe new physics in an area of electroweak scales. And finally, the fact that for some time seemed too strict a restriction - the lightest of the Higgs could not be too heavy, or this mechanism would not work at all.
As the Higgs searches continued without much success, the accelerators consistently approached ever closer to the theoretical upper limit of the light Higgs mass in supersymmetric theories. This value was about 135 proton masses, and the details were already dependent on the specific model. If Higgs had not been found on this scale, it would have meant that all the hype around supersymmetry would have remained a hype.
But it turned out differently. The Higgs, found at the LHC, has a mass 125 times the proton. It is possible that we will reach the great synthesis.
At the moment, the answer is still unclear. Signs of the presence of new supersymmetric particles, if they exist, should have been so clearly visible at the LHC that some physicists thought that the chances of opening supersymmetry were much more likely to find Higgs. But everything went wrong. After three years of BAK operation, no signs of supersymmetry are visible. The situation becomes uncomfortable. The lower limits imposed on the masses of supersymmetric partners of ordinary matter are increasing all the time. If they climb too high, the scale of supersymmetry breaking will no longer be close to the electroweak scale, and many of the attractive properties of supersymmetry breaking that solve the hierarchy problem will simply evaporate.
But the hope remains, and the LHC was switched on again, already at a higher energy. It is possible that supersymmetric particles will soon be detected.

Their discovery will lead to another important result. One of the biggest mysteries of cosmology is the nature of dark matter, which seems to dominate all the galaxies we see. It exists so much that it cannot consist of the same particles as normal matter. If it consisted of normal particles, then, for example, predictions about the abundance of light elements such as helium, obtained during the Big Bang, would not be consistent with observations. Therefore, physicists are fairly confident that dark matter consists of a new type of elementary particles. But what?
The lightest supersymmetric partner of ordinary matter is completely stable in most models, and its properties are in many ways similar to neutrinos. He would interact weakly and was electrically neutral, so he would not absorb and emit light. Moreover, the calculations of 30 years ago, which I was engaged in, showed that the residual abundance of the lightest supersymmetric particle left after the Big Bang would naturally be such that it would correspond to the dominance of dark matter in galaxies.
In this case, our galaxy would have a halo of dark matter particles, with a whistling sound sweeping through its parts, including the room in which you read it. As many of us have long understood, this means that it is possible to develop sensitive underground detectors, similar, at least in essence, to existing neutrino detectors, and to register dark matter particles directly. A dozen wonderful experiments all over the world are trying to do just that. But so far no one has found anything.
Therefore, we now live either in the best or in the worst of times. There is a race between the BAC and underground dark matter detectors for the right to be the first to discover its nature. If one of the groups announces its discovery, it will usher in the discovery of a whole new world of discoveries, potentially leading to an understanding of the TVO itself. And if there are no discoveries in the coming years, it will be possible to exclude the variant of the supersymmetric origin of dark matter - and in general the whole idea of supersymmetry as a solution to the problem of hierarchy. In this case, you will have to go back to the board, however, in the absence of any signals from the LHC, we will not have an idea about which way we will move to develop a new model of nature.
Everything became much more interesting when a possible signal was discovered at the LHC, which promises us a new particle six times heavier than the Higgs. The characteristics of this particle did not match the expected characteristics of any of the supersymmetric partners of ordinary matter. Usually, when processing a large array of data, the most tempting hints of signals disappear, and six months after the onset of this signal and data processing, it disappeared. Otherwise, he could change our entire understanding of the TVW and electroweak symmetry, hint at the existence of a new fundamental interaction and at a new set of particles associated with it. But, although it led to the emergence of hope-inspiring theoretical scientific works, nature decided in its own way.
But one team of physicists does not bother with the absence of a clear experimental detection or confirmation of supersymmetry for a long time and until now. The mathematical beauty of sumersymmetry in 1984 inspired scientists to resurrect an idea that had been dormant since the 1960s. Then Yoichiro Nambu and his colleagues tried to understand the strong interaction, presenting it as string-like signals connecting the quarks. And when supersymmetry was included in quantum string theory to get what became known as superstring theory, amazingly beautiful mathematical results began to appear. Among them is the possibility of combining not only three non-gravitational interactions, but in general all four forces known in nature into one consistent theory of a quantum field.
However, this theory requires the existence of a whole heap of additional dimensions of space-time, which no one has yet observed. In addition, the theory makes no predictions that could be tested at the current experimental level. In addition, it has recently become so complicated that now it seems that the strings themselves are no longer the central dynamic variables of this theory.
But this did not muffle the enthusiasm of the most zealous, devoted and extremely talented adherents of the theory of superstrings, now known as M-theories, who for 30 years continue to work on it. Periodically, there are claims of tremendous success, but so far M-theory lacks a key element that triumphs for such a scientific enterprise as the Standard Model: the ability to get in touch with the world, which we can measure, solve unexplainable riddles, and provide fundamental an explanation of why our world is as it is. This does not mean that the M-theory is erroneous, but so far it represents only reasoning, albeit with a good motive.
If you follow the lessons of history, it becomes clear that most of the advanced physical theories turned out to be false. Otherwise, anyone would be able to do theoretical physics. It took several centuries, and if we take into account the science of the ancient Greeks, then several thousand years, on trial and error, which led to the emergence of the Standard Model.
And here we are. Will new experimental revelations await us in the near future, which will be able to confirm or disprove the greatest arguments of theoretical physics? Or are we on the edge of the desert, and nature will not give us hints about the direction of finding answers to the secrets of space? We will find out, and in any case, we will have to live with a new reality.
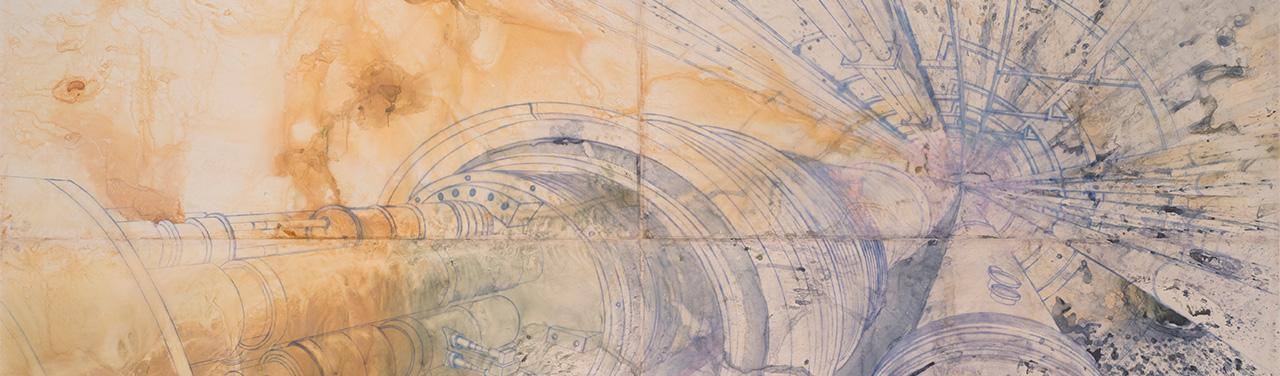
Specialists in particle physics before the discovery of the Higgs particle in 2012 dreamed of two types of nightmares. The first is that the Large Hadron Collider (LHC) will not find anything. In this case, it would be the last major accelerator built to probe the fundamental structure of the universe. The second is that the Higgs particle predicted by theoretical physicist Peter Higgs in 1964 will be found at the LHC ... and nothing more.
Each discovery of one level of reality shows us the following levels. Therefore, each important discovery in science usually leaves us with more questions than answers. But on the other hand, it usually gives us at least an outline of a further path, helping us to find answers to new questions. The successful discovery of the Higgs particle and the confirmation of the existence in space of an invisible background of the Higgs field (in the quantum world, each particle, such as the Higgs particle, is associated with the field), was a powerful confirmation of the bold scientific discoveries of the 20th century.

But the words of Sheldon Lee Glashow have not lost their relevance: the Higgs particle is similar to the sewer. She hides all the untidy details that we don’t want to talk about. The Higgs field interacts with most elementary particles when they move in space, and creates a resistance force that slows their movement and gives them the appearance of mass. Therefore, the masses of elementary particles, measured by us, and making possible our familiar world, are something like an illusion, an accident of our perception.
This idea may look elegant, but in reality it represents a special addition to the Standard Model of Physics - explaining three of the four known forces, and how they interact with matter. It was added to the theory in order to satisfy the requirements for an accurate description of our world. But the theory itself does not require it. The universe could easily exist with massless particles and long-range weak interactions (one of the four interactions — the rest will be strong, electromagnetic, and gravitational force). Just there would not be us and our questions. Moreover, the exact physics of the Higgs model is not defined within the Standard Model alone. A particle could be 20 times heavier or 100 times lighter.
So why does it even exist? And why is she so heavy? (Considering that when a scientist asks the question “Why?”, In fact he means “How?”) If there were no Higgs particles, there would not be such a world as we observe - but this is clearly not possible an explanation. Or can it? To understand the basis of Higgs physics is to understand how we appeared. When we ask: “Why are we here?”, In essence, we ask: “Why is the Higgs here?” And the Standard Model cannot answer this question.
There are some hints derived from a combination of theory and experiment. Shortly after establishing the clear structure of the Standard Model, in 1974, and long before experimentally confirming its details in the next decade, two different groups of Harvard physicists, in which Sheldon Lee Glashow worked, andSteven Weinberg , noticed something interesting. Glashow, together with Howard Georgie , did what he could best: he looked for patterns in existing particles and interactions, and new possibilities with the help of the mathematical theory of groups.
In the Standard Model, weak and electromagnetic interactions are combined at high energies into a single force, which physicists call "electro-weak." This means that the same mathematics controls the weak and electromagnetic interaction, they both obey the same symmetries, and these two forces are different reflections of the same unified theory. But the symmetry is “spontaneously broken” by the Higgs field interacting with particles that undergo weak interaction, but not with particles that transfer electromagnetic. This property of nature leads to the fact that these two interactions look separate and differ on the scales available to our measurements - while the weak interaction works at short distances, and the electromagnetic interaction - at long distances.
Georgie and Glashow attempted to expand this idea and connect strong interactions to them, and found that all known particles and three non-gravitational interactions fit naturally in one fundamentally symmetric structure. They reasoned that this symmetry can spontaneously break down on some ultra-high energy scale (and at a short distance), beyond the limits of the possibilities of modern experiments, and generate two separate symmetries — strong and electro-weak interaction. As a result, at lower energies and at large distances electroweak symmetry is destroyed, dividing the electroweak interaction into weak, acting at short distances, and electromagnetic, acting at long distances.
Such a theory they modestly called the theory of the great unification (TVO).
Around the same time, Weinberg and Georgie, along with Helen Quinn, noticed something interesting in developing the work of Frank Wilcheck, David Gross and David Politzer. If at small distances the strong interaction becomes weaker, then the electromagnetic and weak ones become stronger.
It was not necessary to be a rocket scientist in order to be interested in whether the strength of three different interactions on some small scale does not coincide. After calculating, they found (with the accuracy with which interactions were measured) that such a combination is possible, but only at distances of 15 orders of magnitude smaller than the size of the proton.
If TVO was the one proposed by Howard Georgie and Glashow - then it was good news, because if all the particles we observe in nature combine in this way, then new particles ( gauge bosons ) must exist that provide the connection between the quarks (of which the protons and neutrons) and electrons with neutrinos. This would mean that protons can decay into lighter particles, which we can observe in principle. As Glashow wrote, "diamonds are not forever."
And even then it was known that the proton lifetime was extremely long. Not only because we still exist 14 billion years after the Big Bang, but also because we don’t die of cancer in childhood. If the average lifetime of a proton would be less than a billion billion years, then in childhood enough protons would decay in our body so that their radiation would kill us. In quantum mechanics, all processes are probabilistic. If the average proton lives a billion billion years, and if you have a billion billion protons, then one of them will decay on average every year. And in our body is much more than a billion billion protons.
However, with such an incredibly small scale of distances, and, therefore, with such a huge mass scale associated with spontaneous symmetry breaking in TVO, the new gauge bosons receive huge masses. And this would lead to the fact that the interactions controlled by them would occur at such small distances that they would be incredibly weak in terms of protons and neutrons. As a result, although the protons may decay, in our case, before that, they can live, perhaps a million billion billion billion years.
Thanks to the results obtained by Glashow with Georgie, as well as Georgie with Quinn and Weinberg, the expectations of the great synthesis were in the air. After the success of the electroweak theory of physics, studying particles, were ambitious and believed in the subsequent unification.
How do you know if these ideas are true? It was impossible to build an accelerator capable of working on energies a million billion times more than the rest mass of protons. The circumference of such a machine would have to be compared with the orbit of the moon. And even if it were possible, as a result of the fiasco of the Superconducting supercollider, no government would approve such an estimate. [This collider, also called the Desertron, should have been built in Texas in the 1990s, but due to problems with the budget was cancelled. It was planned that the length of its circumference will be 87.1 km. $ 2 billion was spent on construction, and the final cost was estimated at $ 12 billion - approx. trans.].
Fortunately, there was another way - to use the probability described by me, which limits the proton lifetime. If TVO predicts a proton lifetime of one thousand billion billion billion years, then you need to push a thousand billion billion billion protons into one detector, and then on average one of them will decay every year.
And where do you get so many protons? It's simple: in 3000 tons of water.
All that was required for that was to place a tank of water in the dark, make sure that there was no radioactive background in this place, surround it with sensitive photocells able to detect flashes of light in the detector, and then wait a year waiting for a flash of light when a proton decays. It sounds scary, but at least two large experimental facilities were paid for and built according to this scheme - one deep underground in the salt mine near Lake Erie (IMB), the other in the Kamioka zinc mine in Japan (Kamiokanda). The mines were used to cut off cosmic rays, against which it would have been impossible to notice the proton decay.

The Large Hadron Collider
Both experiments began to work in 1982-1983. Scientists were so enthusiastic about TVO that they were confidently waiting for the signal to appear soon. In this case, a TSS would be the culmination of a decade of tremendous development and discoveries in particle physics - not to mention the next Nobel Prize for Glashow , and possibly some more.
Unfortunately, in this case, nature was not so good. Not a single signal appeared either in the first year, or in the second, or in the third. The simplest and elegant model of Glashow and Georgie soon had to be rejected. But the fever TVO has already captured scientists, and it was difficult to get rid of it. Other proposals were made about theories of unification, because of which the decay of protons would go beyond the framework of current experiments.
On February 23, 1987, another event happened that again demonstrated an almost universal aphorism: every new window into the Universe takes us by surprise. On that day, a group of astronomers on photographic plates accumulated overnight discovered the nearest exploded star (supernova) of all that we have seen in the last 400 years. This star, which was 160,000 light-years away, was in the Large Magellanic Cloud — a small galaxy, a satellite of the Milky Way, which can be seen in the southern hemisphere.
If our theories about exploding stars are correct, most of the energy they emit should take the form of neutrinos, despite the fact that the light of their explosion is the brightest of cosmic fireworks (and they explode about one star in one galaxy in 100 years). Rough calculations showed that the IMB and Kamiokande water detectors were to detect about 20 collisions with neutrinos. And when the experimenters of these detectors studied the data of that day, 8 candidates were found at IBM on a 10-second interval, and on Kamiokand - 11. For neutrino physics, this was just a sea of data. Neutrino astrophysics has suddenly matured. Probably 1900 scientific works of various physicists (including me) were based on these 19 events. They realized that this event opened an unprecedented window into the nuclei of exploding stars, and into the laboratory not only for astrophysics,
Driven by the idea that large proton decay detectors can simultaneously become astrophysical neutrino detectors, several groups of scientists have begun to build a new generation of such dual-use detectors. The largest was re-built in the Kamioka mine and dubbed Super-Kamiokande - and for good reason. This giant reservoir of water weighing 50,000 tons, surrounded by 11,800 photocells, worked in an operating mine, and the experiment was conducted with laboratory cleanliness. It was necessary, because with such a huge detector it was necessary to take care not only of external cosmic rays, but also of internal radioactive contamination, which would eclipse all useful signals.
Meanwhile, interest in astrophysical neutrinos was also at its peak. The sun emits neutrinos as a result of nuclear reactions in its core, and for 20 years the physicist Ray Davis discovered solar neutrinos, but the events happened three times less often than the best models of the Sun predicted. A new type of solar neutrino detector, called the Sudbury Neutrino Observatory (SNO), was built in the mine town of Sudbury in Canada.
To date, the Super-Kamiokande has almost continuously worked, sometimes undergoing various improvements, for 20 years. No signals from the decay of protons and no new supernovae have been observed since. However, accurate neutrino observations, coupled with additional observations at SNO, unequivocally confirmed the reality of the solar neutrino deficit, discovered by Ray Davis. It was found that the deficit does not exist because of astrophysical phenomena occurring in the Sun, but because of the properties of the neutrino. It became clear that at least one of the three types of neutrinos is not massless. Since the Standard model of the neutrino mass is not included, it was the first confirmed observation that some new physics in nature works outside the Standard Model and the Higgs.
High-energy neutrinos regularly bombard the Earth after protons from high-energy cosmic rays collide with the atmosphere and produce a wide air shower from secondary subatomic particles, where these neutrinos are also encountered. Observations on them showed that the second type of neutrino has a mass. It is slightly larger than the first, but much smaller than the electron mass. For this observation, teams from SNO and Kamiokande received the Nobel Prize in 2015 - a week before I started writing this book. To this day, these seductive hints of new physics have not been explained with the help of our theories.
The lack of proton decay was a disappointment, but not a complete surprise. From the moment when TWR was first proposed, the landscape of physics has changed. More accurate measurements of the values of three non-gravitational interactions, together with more complex calculations of changing their magnitude with distance, showed that if only particles from the Standard Model exist in nature, then the forces of these three interactions will not unite on the same scale. In order for the Great Unification to occur, there will have to be a new physics on an energy scale that surpasses everything we have seen so far. And the presence of new particles would not only change the energy scale for combining the three interactions, but also increase the scale for TVO,

The Large Hadron Collider.
In parallel with these events, theorists actively used new mathematical tools to study a new probable type of symmetry, which they began to call supersymmetry. This fundamental symmetry differs from other previously known ones in that it connects two different types of particles — fermions (particles with a half-integer spin) and bosons (particles with a whole spin). But the point is that if such symmetry is observed in nature, then for every particle known in the Standard Model there must be at least one new particle. For each new boson, a fermion must exist. For each fermion is a boson.
Since we do not observe these particles, this symmetry cannot manifest itself at the level of the universe accessible to us, and, therefore, must be broken - and this means that new particles must have masses large enough for them not to be found in existing accelerators.
What is so attractive about symmetry, which suddenly doubles the number of particles in nature, when there is no evidence of their existence? For the most part, she seduces with Great Unification. Since if TVO exists on the scale of masses by 15-16 orders of magnitude of a large rest mass of a proton, then it is 13 orders of magnitude larger than the scales on which electroweak symmetry is broken. The question is how and why in the fundamental laws of nature there is such a huge scale gap. In particular, if the Higgs is really the last particle of the Standard Model, then the question arises: why is the energy scale of the violation of the Higgs symmetry by 13 orders of magnitude smaller than the scale of violation of the symmetry of some new field that breaks the TVO symmetry into separate interactions?
And the problem is even worse than it seems. If we consider the effects of virtual particles (arising and disappearing so quickly that their existence can only be confirmed indirectly), including particles of arbitrarily large mass (such as the gauge particles of the proposed TVO), it becomes clear that the mass and scale of the Higgs symmetry violation increase so that approaching the huge scale of TVO. From here comes the so-called. the problem of naturalness . Technically speaking, there is an unnatural existence of a huge hierarchy of scales , from those on which the Higgs particle breaks electroweak symmetry, and to those on which the symmetry of TVO is broken by a new scalar field, whatever that may be.
Mathematical physicist Edward Whitten wrote in his landmark work of 1981 that supersymmetry has a special property. It can reduce the effect of virtual particles of arbitrarily large mass and energy on the properties of the world on the scales that are available for our observations. Since virtual fermions and virtual bosons of the same mass lead to identical, not counting the sign, quantum corrections, if each boson corresponds to a fermion of the same mass, then the quantum effects of virtual particles are mutually destroyed. And this means that the effect of virtual particles of arbitrarily large mass and energy on the properties of the world on the scales that are available for our observations completely disappear.
If supersymmetry itself is broken (as it should be, otherwise all supersymmetric partners of ordinary matter would have the same mass as ordinary particles, and we would have discovered them long ago), then quantum corrections are not destroyed. They lead to additions to masses on scales comparable to those on which supersymmetry is broken. If they were comparable to the scale of breaking of electroweak symmetry, this would explain the scale of the Higgs mass.
This also means that we can expect to see a heap of new particles — supersymmetric partners of ordinary matter — on the scales that the LHC is now probing.
This would solve the problem of naturalness, since it would protect the Higgs boson masses from quantum corrections that would inflate them to the energy scale of the TWR. Supersymmetry can allow the existence of a “naturally” large hierarchy of energy and mass, which separates the electro-weak scale and the scale of TVO.
The fact that supersymmetry can in principle solve the problem of hierarchy has increased its appeal to physicists. Theorists began to explore realistic models that included the violation of supersymmetry and other consequences of this idea. After that, the “market value” of supersymmetry broke all records. Since, if we include the possibility of spontaneous supersymmetry breaking in the calculations of the change in three non-gravitational interactions depending on the distance, it turns out that the strength of these three interactions naturally suddenly converges to one scale of small distances. TVO returned to duty!
The models with broken supersymmetry have another attractive feature. Long before the discovery of the upper quark, it was shown that if the upper quark is heavy, then through interaction with other supersymmetric partners it can contribute to quantum corrections to the properties of the Higgs particle, which will lead to a change in the Higgs field. If the Grand Unification happens on a much larger energy scale, then the Higgs field should form a coherent background throughout the space at the energy level at which the current measurements are taken. In short, the energy scale of the electroweak symmetry breaking can naturally occur in a theory in which VO happens on a much larger scale. And when the top quark was discovered and found that it was really heavy,
But all this has a price. For a theory to work, there must be two Higgs bosons, not just one. Moreover, in such an accelerator as the LHC, it would be necessary to find new supersymmetric particles — it can probe new physics in an area of electroweak scales. And finally, the fact that for some time seemed too strict a restriction - the lightest of the Higgs could not be too heavy, or this mechanism would not work at all.
As the Higgs searches continued without much success, the accelerators consistently approached ever closer to the theoretical upper limit of the light Higgs mass in supersymmetric theories. This value was about 135 proton masses, and the details were already dependent on the specific model. If Higgs had not been found on this scale, it would have meant that all the hype around supersymmetry would have remained a hype.
But it turned out differently. The Higgs, found at the LHC, has a mass 125 times the proton. It is possible that we will reach the great synthesis.
At the moment, the answer is still unclear. Signs of the presence of new supersymmetric particles, if they exist, should have been so clearly visible at the LHC that some physicists thought that the chances of opening supersymmetry were much more likely to find Higgs. But everything went wrong. After three years of BAK operation, no signs of supersymmetry are visible. The situation becomes uncomfortable. The lower limits imposed on the masses of supersymmetric partners of ordinary matter are increasing all the time. If they climb too high, the scale of supersymmetry breaking will no longer be close to the electroweak scale, and many of the attractive properties of supersymmetry breaking that solve the hierarchy problem will simply evaporate.
But the hope remains, and the LHC was switched on again, already at a higher energy. It is possible that supersymmetric particles will soon be detected.

Their discovery will lead to another important result. One of the biggest mysteries of cosmology is the nature of dark matter, which seems to dominate all the galaxies we see. It exists so much that it cannot consist of the same particles as normal matter. If it consisted of normal particles, then, for example, predictions about the abundance of light elements such as helium, obtained during the Big Bang, would not be consistent with observations. Therefore, physicists are fairly confident that dark matter consists of a new type of elementary particles. But what?
The lightest supersymmetric partner of ordinary matter is completely stable in most models, and its properties are in many ways similar to neutrinos. He would interact weakly and was electrically neutral, so he would not absorb and emit light. Moreover, the calculations of 30 years ago, which I was engaged in, showed that the residual abundance of the lightest supersymmetric particle left after the Big Bang would naturally be such that it would correspond to the dominance of dark matter in galaxies.
In this case, our galaxy would have a halo of dark matter particles, with a whistling sound sweeping through its parts, including the room in which you read it. As many of us have long understood, this means that it is possible to develop sensitive underground detectors, similar, at least in essence, to existing neutrino detectors, and to register dark matter particles directly. A dozen wonderful experiments all over the world are trying to do just that. But so far no one has found anything.
Therefore, we now live either in the best or in the worst of times. There is a race between the BAC and underground dark matter detectors for the right to be the first to discover its nature. If one of the groups announces its discovery, it will usher in the discovery of a whole new world of discoveries, potentially leading to an understanding of the TVO itself. And if there are no discoveries in the coming years, it will be possible to exclude the variant of the supersymmetric origin of dark matter - and in general the whole idea of supersymmetry as a solution to the problem of hierarchy. In this case, you will have to go back to the board, however, in the absence of any signals from the LHC, we will not have an idea about which way we will move to develop a new model of nature.
Everything became much more interesting when a possible signal was discovered at the LHC, which promises us a new particle six times heavier than the Higgs. The characteristics of this particle did not match the expected characteristics of any of the supersymmetric partners of ordinary matter. Usually, when processing a large array of data, the most tempting hints of signals disappear, and six months after the onset of this signal and data processing, it disappeared. Otherwise, he could change our entire understanding of the TVW and electroweak symmetry, hint at the existence of a new fundamental interaction and at a new set of particles associated with it. But, although it led to the emergence of hope-inspiring theoretical scientific works, nature decided in its own way.
But one team of physicists does not bother with the absence of a clear experimental detection or confirmation of supersymmetry for a long time and until now. The mathematical beauty of sumersymmetry in 1984 inspired scientists to resurrect an idea that had been dormant since the 1960s. Then Yoichiro Nambu and his colleagues tried to understand the strong interaction, presenting it as string-like signals connecting the quarks. And when supersymmetry was included in quantum string theory to get what became known as superstring theory, amazingly beautiful mathematical results began to appear. Among them is the possibility of combining not only three non-gravitational interactions, but in general all four forces known in nature into one consistent theory of a quantum field.
However, this theory requires the existence of a whole heap of additional dimensions of space-time, which no one has yet observed. In addition, the theory makes no predictions that could be tested at the current experimental level. In addition, it has recently become so complicated that now it seems that the strings themselves are no longer the central dynamic variables of this theory.
But this did not muffle the enthusiasm of the most zealous, devoted and extremely talented adherents of the theory of superstrings, now known as M-theories, who for 30 years continue to work on it. Periodically, there are claims of tremendous success, but so far M-theory lacks a key element that triumphs for such a scientific enterprise as the Standard Model: the ability to get in touch with the world, which we can measure, solve unexplainable riddles, and provide fundamental an explanation of why our world is as it is. This does not mean that the M-theory is erroneous, but so far it represents only reasoning, albeit with a good motive.
If you follow the lessons of history, it becomes clear that most of the advanced physical theories turned out to be false. Otherwise, anyone would be able to do theoretical physics. It took several centuries, and if we take into account the science of the ancient Greeks, then several thousand years, on trial and error, which led to the emergence of the Standard Model.
And here we are. Will new experimental revelations await us in the near future, which will be able to confirm or disprove the greatest arguments of theoretical physics? Or are we on the edge of the desert, and nature will not give us hints about the direction of finding answers to the secrets of space? We will find out, and in any case, we will have to live with a new reality.