
Transistor Story Part 2: From the Crucible of War
- Transfer

Other articles in the series:
- Relay history
- The history of electronic computers
- Transistor history
- Internet history
The crucible of war paved the way for the appearance of a transistor. From 1939 to 1945, technical knowledge in the field of semiconductors grew incredibly. And there was one simple reason: radar. The most important technology of the war, among the applications of which are: air raid detection, search for submarines, sending night flights to targets, guidance of air defense systems and sea guns. Engineers even learned to shove tiny radars into artillery shells so that they explode when flying near the target - radio fuses . However, the source of this powerful new military technology was a more peaceful area: the study of the upper atmosphere for scientific purposes.
Radar
In 1901, Marconi Wireless Telegraph successfully transmitted wireless communications across the Atlantic from Cornwall to Newfoundland. This fact has confused modern science. If the radio transmits in a straight line (as it should be), such a transmission should be impossible. There is no direct line of sight between England and Canada that does not cross the Earth, so Marconi's message was supposed to fly into space. American engineer Arthur Kenneli and British physicist Oliver Heaviside both simultaneously and independently suggested that the explanation for this phenomenon should be related to a layer of ionized gas located in the upper atmosphere that can reflect radio waves back to the Earth (Marconi himself believed that radio waves follow the curvature of the Earth’s surface, however, physicists did not support him).
By the 1920s, scientists had developed new equipment that allowed them to first prove the existence of the ionosphere, and then study its structure. They used electron tubes to generate shortwave radio pulses, directional antennas to send them up into the atmosphere and record echoes, and cathode-ray devices to demonstrate the results. The longer the echo return delay, the farther the ionosphere should be. This technology was called atmospheric sounding, and it provided the basic technical infrastructure for creating the radar (the term "radar", from RAdio Detection And Ranging, only appeared in the US Navy in the 1940s).
The fact that people with the necessary knowledge, resources and motivation, understood the potential of ground-based use of such equipment was only a matter of time (thus, the history of the radar is opposite to the history of the telescope, which was originally intended for ground use). And the likelihood of such an illumination increased as the radio spread more and more around the planet, and more and more people noticed interference coming from nearby ships, planes and other large objects. Upper atmosphere sounding knowledge spread during the second International Polar Year(1932-1933), when scientists from different Arctic stations compiled a map of the ionosphere. Soon after this, teams in Britain, the USA, Germany, Italy, the USSR and other countries developed their simplest radar systems.

Robert Watson-Watt with his 1935 radar
Then there was a war, and the importance of radars to countries - and the resources to develop them - increased dramatically. In the United States, these resources have gathered around a new organization, founded in 1940 at MIT, known as Rad Lab.(It was named so specifically to mislead foreign spies, and create the impression that the laboratory was investigating radioactivity - then few people believed in atomic bombs). The Rad Lab project, not so famous as the Manhattan project, nevertheless got into its ranks the same outstanding and talented physicists from all over the USA. Five of the first laboratory staff (including Luis Alvarez and Isidore Isaac Rabi) subsequently received the Nobel Prizes. By the end of the war, about 500 doctors of science, scientists and engineers were working in the laboratory, and a total of 4,000 people worked. Half a million dollars - which is comparable to the full ENIAC budget - was spent only on the Radiation Laboratory Series, twenty-seven volumes, which described all the knowledge gained in the laboratory during the war (while the US government spent on radar technology was not limited to the Rad Lab budget ; during the war, the government purchased $ 3 billion in radar).

20th MIT building, where Rad Lab was located
One of Rad Lab’s main research areas has been high-frequency radar. Early radars used waves with lengths measured in meters. But rays of a higher frequency, the wavelengths of which were measured in centimeters - microwaves - made it possible to use more compact antennas and scattered less at large distances, which promised great advantages in range and accuracy. Microwave radars could fit in the nose of an airplane and detect objects the size of a periscope of a submarine.
The first to solve this problem was a team of British physicists from the University of Birmingham. In 1940 they developed the " resonant magnetron"that worked like an electromagnetic" whistle ", turning a random pulse of electricity into a powerful and finely tuned microwave beam. This microwave transmitter was a thousand times more powerful than its closest competitor; it paved the way for practical high-frequency radar transmitters. However, it required companion, a receiver capable of recording high frequencies. And at this point we return to the story of semiconductors.
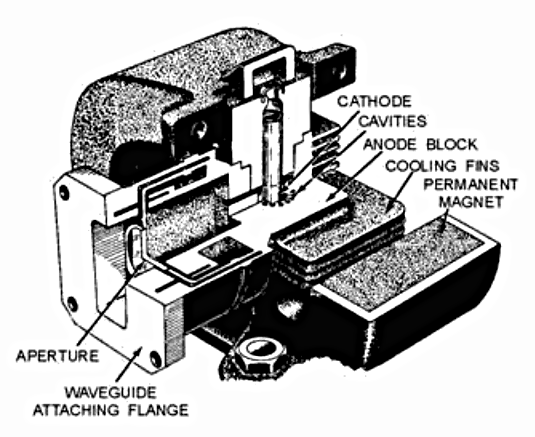
magnetron sectional
The Second Coming of the Cat Mustache
It turned out that electron tubes were not at all adapted to receive microwave radar signals. The gap between the hot cathode and the cold anode creates a capacitance, because of which the circuit refuses to work at high frequencies. The best technology for the high-frequency radars available was the old-fashioned cat whisker , a small piece of wire pressed against a semiconductor chip. This was independently discovered by several people, but what happened in New Jersey is closest to our history.
In 1938, Bell’s laboratories signed a contract with the Navy to develop a fire control radar in the 40 cm range - it was much shorter, and therefore more frequency, than the radars existing then in the era before resonant magnetrons. The main research work was received by the laboratory division in Holmdel, south of Staten Island. Researchers did not take a long time to figure out what they would need for a high-frequency receiver, and soon engineer George Southworth scoured radio stores in Manhattan in search of old cat-mustache detectors. As expected, it worked much better than a lamp detector, but it was unstable. So Southworth tracked down an electrochemist named Russell All, and asked him to try to improve the uniformity of the response of a crystalline detector with a single point of contact.
Ol was a rather peculiar person, who considered the development of technology to be his fate, and spoke about periodic inspirations with visions of the future. For example, he stated that as early as 1939 he knew about the future invention of a silicon amplifier, but that fate was destined to invent it for another person. Having studied dozens of options, he settled on silicon as the best substance for Southworth receivers. The problem was the ability to control the contents of the material in order to control its electrical properties. Then, industrial silicon blanks were widely distributed, they were used in steel mills, but nobody bothered in such a production, for example, the content of 1% phosphorus in silicon. Enlisting the help of a couple of metallurgists, Ol set out to get much cleaner discs than previously possible.
In the process, they found that some of their crystals rectified current in one direction and others in the other. They called them "n-type" and "p-type." Further analysis showed that different types of impurities were responsible for these types. Silicon is in the fourth column of the periodic table, that is, it has four electrons on the outer shell. In a blank of pure silicon, each of these electrons would unite with a neighbor. The impurities from the third column, for example, boron, which has one less electron, created a “hole”, additional space for the current to flow in the crystal. The result was a p-type semiconductor (with an excess of positive charges). Elements from the fifth column, for example, phosphorus, provided additional free electrons for carrying current, and an n-type semiconductor was obtained.

Crystal structure of silicon
All these studies were very interesting, but by 1940 Southworth and Ol did not come close to creating a working prototype of a high-frequency radar. The British government at the same time demanded immediate practical results due to the impending threat from the Luftwaffe, where they had already created ready-for-production microwave detectors working in conjunction with magnetron transmitters.
However, soon the balance of technological advances will lean towards the western side of the Atlantic. Churchill decided to reveal all the technical secrets of Britain to the Americans before he actually entered the war (since, as he assumed, this was to happen anyway). He believed that it was worth the risk of an information leak, since then all the industrial capabilities of the United States would be thrown into solving problems such as atomic weapons and radars. The British scientific and technical mission (better known as the Tizard mission ) arrived in Washington in September 1940 and brought in a luggage a gift in the form of technical miracles.
Revealing the incredible power of a resonant magnetron and the effectiveness of British crystal detectors in receiving its signal has revitalized the research of Americans in the field of semiconductors as the basis of high-frequency radars. There was a lot of work to be done, especially in the field of materials science. To meet the demands, semiconductor crystals “were required to be produced in millions, much more than previously possible. It was necessary to improve the straightening, reduce the sensitivity to shock and the probability of burnout, and minimize the difference between different batches of crystals. "

Silicon Point Rectifier
Rad Lab has opened new research departments to study the properties of semiconductor crystals and how they can be changed to maximize valuable properties as a receiver. The most promising materials were silicon and germanium, so Rad Lab decided to play it safe and launched parallel programs to study both: silicon at the University of Pennsylvania, and germanium at Purdue. Industrial giants such as Bell, Westinghouse, Du Pont and Sylvania have begun their own semiconductor research programs, and have begun developing new manufacturing facilities for crystal detectors.
Together, the purity of the crystals of silicon and germanium was raised from 99% at the beginning to 99.999% - that is, to one impurity particle per 100,000 atoms. In the process, the cadre of scientists and engineers became closely acquainted with the abstract properties of germanium and silicon and applied technologies for controlling them: melting, crystal growth, adding the necessary impurities (such as boron, which increased conductivity).
And then the war ended. Demand for radars has disappeared, but the knowledge and skills gained during the war have not gone away, and the dream of a solid-state amplifier has not been forgotten. Now the race was to create such an amplifier. And at least three teams were in a good position to receive this prize.
West Lafayette
The first was a group from Purdue University led by a physicist of Austrian descent named Karl Lark-Horowitz. Using talent and influence, he single-handedly removed the university’s physics department from oblivion and influenced Rad Lab’s decision to entrust his laboratory with germanium research.
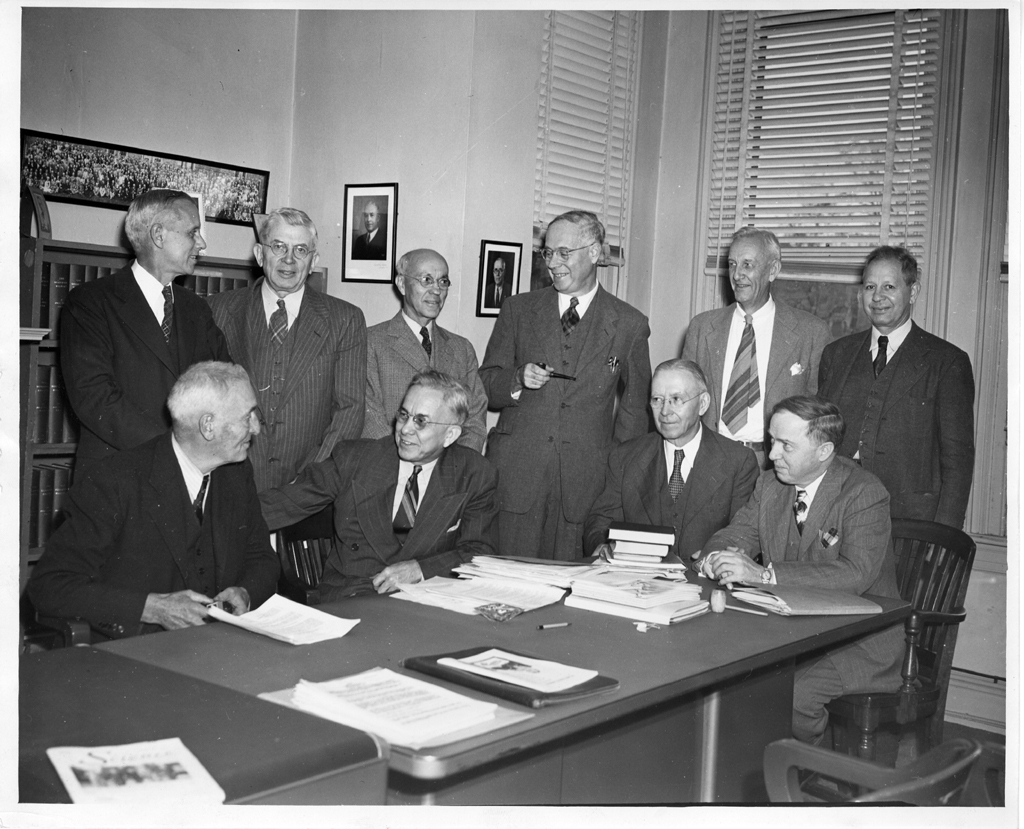
Karl Lark Horowitz in 1947, in the center, with a pipe
By the early 1940s, silicon was considered the best material for radar rectifiers, but the material directly below it in the periodic table also looked worthy of further study. Germany had a practical advantage due to its lower melting point, which made it easier to work with it: about 940 degrees, compared to 1400 degrees for silicon (almost like steel). Due to the high melting point, it was extremely difficult to make a pig that would not leak into molten silicon, contaminating it.
Therefore, Lark-Horowitz and his colleagues spent the entire war studying the chemical, electrical, and physical properties of Germany. The most important obstacle was the “reverse voltage”: germanium rectifiers at very low voltage ceased to rectify the current and allowed it to flow in the opposite direction. A reverse current pulse burned the remaining components of the radar. One of Lark Horowitz’s graduate students, Seymour Benzer, studied this problem for more than a year, and finally developed a tin-based additive that stopped reverse pulses at voltages up to hundreds of volts. Shortly thereafter, Western Electric, the manufacturing unit of Bell's laboratory, began to produce rectifiers based on the Benzer circuit for military purposes.
The study of Germany in Purdue continued after the war. In June 1947, Benzer, already a professor, reported an unusual anomaly: in some experiments, high-frequency vibrations appeared in germanium crystals. And his colleague Ralph Bray continued to study the “volumetric resistance” of the project, which began during the war. Volumetric resistance described how electricity flows in a germanium crystal at the contact point of a rectifier. Bray found that high voltage pulses significantly reduced the resistance of n-type germanium to these currents. Unaware of this, he witnessed the so-called “Minority” charge carriers. In n-type semiconductors, excess negative charge serves as the main charge carrier, but positive “holes” can also carry current, and in this case, high-voltage pulses created holes in the germanium structure,
Bray and Benzer came seductively close to the Germanium amplifier, not realizing it. Benzer caught Walter Brattain, a scientist from Bell’s laboratories, at a conference in January 1948 to discuss volumetric resistance with him. He suggested that Brattain arrange another point contact next to the first, which could conduct current, and then they might be able to understand what is happening under the surface. Brattain quietly agreed with this proposal, and left. As we shall see, he knew too well what a similar experiment could reveal.
One sous bois
The Purdue group had both the technology and the theoretical basis for making the leap towards the transistor. But they could stumble upon it only by chance. They were interested in the physical properties of the material, and not in the search for a new type of device. A completely different situation reigned in Onet-sous-Bois (France), where two former German radar researchers, Heinrich Welker and Herbert Mathare, led a team whose goal was to create industrial semiconductor devices.
Velker first studied and then taught physics at the University of Munich, managed by the famous theorist Arnold Sommerfeld. Since 1940, he left a purely theoretical path and began working on a radar for the Luftwaffe. Matar (of Belgian origin) grew up in Aachen, where he studied physics. He joined the research department of the German radio giant Telefunken in 1939. During the war, he transferred his work from Berlin east to the abbey in Silesia to avoid anti-Hitler coalition air raids, and then back west to avoid the advancing Red Army, and eventually fell into the hands of the American army.
Like their rivals from the Anti-Hitler coalition, the Germans by the early 1940s knew that crystal detectors were ideal receivers for radars, and that silicon and germanium were the most promising materials for their creation. Matera and Velker during the war tried to improve the effective use of these materials in rectifiers. After the war, both underwent periodic interrogations regarding their military work, and eventually received an invitation from the French intelligence officer to Paris in 1946.
Compagnie des Freins & Signaux (the “company of brakes and signals”), the Westinghouse French division, received a contract from the French telephone company for the development of solid state rectifiers and was looking for German scientists to help themselves. Such a union of recent enemies may seem strange, but this arrangement has proved to be quite favorable for both sides. The French, defeated in 1940, did not have the opportunity to gain knowledge in the field of semiconductors, and they desperately needed the skills of the Germans. The Germans could not conduct development in any high-tech areas in the country occupied and destroyed by the war, so they seized on the possibility of continuing work.
Velker and Mathare set up a headquarters in a two-story house in the suburbs of Paris, One-sous-Bois, and with the help of a team of technicians arranged the successful production of germanium rectifiers by the end of 1947. Then they turned to more serious prizes: Velker returned to the superconductors he was interested in, and Matar amplifiers.

Herbert Mathare in 1950
During the war, Matera experimented with rectifiers with two point contacts - “duodiodes” - in an attempt to reduce noise in the circuit. He resumed his experiments and soon discovered that the second “cat's whisker,” located 1/100 million of a meter from the first, could sometimes modulate the current passing through the first whisker. He created a solid-state amplifier, although rather useless. To achieve a more reliable operation, he turned to Velker, who had gained extensive experience working with germanium crystals during the war. The Velker team grew larger and cleaner samples of germanium crystals, and along with improved material quality, by June 1948, Matera point contact amplifiers had become reliable.

X-ray image of the "transistron" based on the Matare scheme, which has two points of contact with germanium
Matera even had a theoretical model of what was happening: he believed that the second contact made holes in germany, accelerating the passage of current through the first contact, supplying minority charge carriers. Velker did not agree with him, and believed that what was happening depended on a certain field effect. However, before they could work out a device or theory, they learned that a group of Americans had developed exactly the same concept - a Germanium amplifier with two point contacts - six months earlier.
Murray Hill
At the end of the war, Mervyn Kelly reformed Bell’s semiconductor research team, led by Bill Shockley. The project expanded, received more funding, and moved from the original laboratory building in Manhattan to an expanding campus in Murray Hill (New Jersey).

Campus in Murray Hill, approx. 1960
To get to know advanced semiconductors again (after he was involved in operations research in the war), in the spring of 1945, Shockley visited the Russell Ola laboratory in Holmdel. Ol spent the war years working on silicon, and did not lose time in vain. He showed Shockley a rude amplifier of his own construction, which he called a "desister." He took a silicon point contact rectifier and ran current from the battery through it. Apparently, the heat of the battery reduced the resistance through the contact point, and turned the rectifier into an amplifier, capable of transmitting incoming radio signals to the circuit, powerful enough to power the speaker
The effect was rude and unreliable, unsuitable for commercialization. However, it was enough to confirm Shockley's opinion about the possibility of creating a semiconductor amplifier, and that this should be made a priority in research in the field of solid-state electronics. Also, this meeting with the Ola team convinced Shockley that silicon and germanium should be studied first. They showed attractive electrical properties, and in addition, Ola’s colleagues, metallurgists Jack Scuff and Henry Terer, achieved tremendous success in growing, refining and adding impurities to these crystals during the war, surpassing all the technologies available for other semiconductor materials. The Shockley group was no longer going to waste time on pre-war copper oxide amplifiers.
With Kelly's help, Shockley began to assemble a new team. Key players included Walter Bretstein, who helped Shockley with his first attempt at creating a semiconductor amplifier (in 1940), and John Bardin, a young physicist and new Bell lab worker. Bardin probably had the most extensive knowledge of solid state physics of all team members — his dissertation described the energy levels of electrons in the structure of metallic sodium. He was also another protagonist of John Hazbrook Van Fleck, like Atanasov and Brettain.
And like Atanasov, the dissertations of Bardin and Shockley required complex calculations. They had to use the quantum-mechanical theory of semiconductors, defined by Alan Wilson, to calculate the energy structure of materials using a Monroe desktop calculator. Helping to create a transistor, they, in fact, contributed to the deliverance of future graduate students from such work.
Shockley's first solid-state amplifier approach relied on what was later called the " field effect "". He hung a metal plate over an n-type semiconductor (with an excess of negative charges). The application of a positive charge to the plate pulled an excess of electrons onto the surface of the crystal, creating a river of negative charges through which electric current could easily flow. An amplified signal (represented by the charge level on plate) in this way could modulate the main circuit (passing over the surface of the semiconductor). The operability of this circuit was prompted to him by his theoretical knowledge in physics. But, despite m ozhestvo tests and experiments, the scheme has not worked.
By March 1946, Bardin had created a well-developed theory that explained the reason for this: the surface of a semiconductor at a quantum level behaves differently from its interiors. Negative charges drawn to the surface fall into the trap of “surface states” and block the penetration of the electric field from the plate into the material. The rest of the team found this analysis convincing, and launched a new research program in three ways:
- Prove the existence of surface states.
- Examine their properties.
- Come up with how to defeat them and make a working field effect transistor .
After a year and a half of research and experimentation, on November 17, 1947, Brettein made a breakthrough. He found that if you place a liquid filled with ions, such as water, between the wafer and the semiconductor, the electric field from the wafer will push the ions toward the semiconductor, where they will neutralize the charges caught in the surface states. Now he could control the electrical behavior of a piece of silicon, changing the charge on the plate. This success gave Bardin an idea for a new approach to creating an amplifier: to surround the contact point of the rectifier with electrolyte water, and then use a second wire in water to control surface states, and in this way control the level of conductivity of the main contact. So Bardin and Brettain entered the finish line.
Bardin's idea worked, but the gain was weak and worked at very low frequencies, inaccessible to the human ear - therefore it was useless in the role of a telephone or radio amplifier. Bardin proposed switching to reverse-voltage resistant germanium obtained at Purdue, believing that fewer charges would collect on its surface. Suddenly they received a powerful boost, but in the opposite direction from the expected. They discovered the effect of minority carriers - instead of the expected electrons, the current passing through germanium strengthened the holes coming from the electrolyte. The current on the wire in the electrolyte created a p-type layer (the region of excess positive charges) on the surface of n-type germanium.
Subsequent experiments showed that the electrolyte was not needed at all: simply by placing two contact points close to the surface of germanium, it was possible to modulate the current from one of them to the other. To bring them as close as possible, Bretstein wrapped a triangular piece of plastic around a piece of gold foil, and then carefully cut the foil at the end. Then, using a spring, he pressed the triangle to Germany, as a result of which the two edges of the cut touched its surface at a distance of 0.05 mm. This gave the prototype of the transistor from Bell's laboratories its distinctive appearance:

The prototype of the Bretstein and Bardin transistor
Like the Matare and Velker device, it was, in principle, a classic “feline mustache”, just with two points of contact instead of one. On December 16, he issued a significant power and voltage gain, and a frequency of 1000 Hz in the range of audibility. A week later, after small improvements, Bardin and Bretstein received a voltage increase of 100 times and a power of 40 times, and showed Bell's directors that their device can reproduce audible speech. John Pearce, another member of the solid-state device development team, coined the term “transistor” based on the name for a copper oxide rectifier, a varistor.
The next six months, the laboratory kept the new creation a secret. The management wanted to make sure that they would have a head start in realizing the commercial capabilities of the transistor before anyone else got it. A press conference was scheduled for June 30, 1948, just in time to shatter all of Velker's and Matara's dreams of immortality. Meanwhile, the semiconductor research team quietly fell apart. Hearing about the achievements of Bardin and Bretstein, their boss, Bill Shockley, began to work to take over all the fame. And although he played only an observant role, in a public presentation, Shockley received equal, if not large, advertising - as can be seen from this published picture, where he is in the thick of things, and right at the laboratory table:

Advertising photo of 1948 - Bardin, Shockley and Bretstein
However, Shockley was not of equal fame. And even before anyone outside of Bell’s labs found out about the transistor, he set about re-inventing it to appropriate it. And this was only the first of many such repeated inventions.
What else to read
- Robert Buderi, The Invention That Changed the World (1996)
- Michael Riordan, “How Europe Missed the Transistor,” IEEE Spectrum (Nov. 1, 2005)
- Michael Riordan and Lillian Hoddeson, Crystal Fire (1997)
- Armand Van Dormael, “The 'French' Transistor,” www.cdvandt.org/VanDormael.pdf (1994)